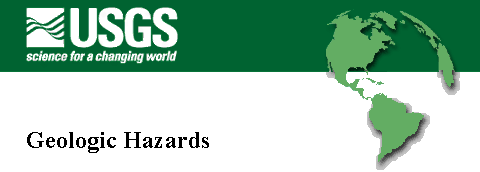
PRE-1980 TEPHRA-FALL DEPOSITS ERUPTED FROM MOUNT ST. HELENS, WASHINGTON
By Donal R. Mullineaux
U. S. Geological Survey Professional Paper 1563
ERUPTIVE HISTORY
Mount St. Helens is much younger and has been more explosively active recently
than other major Cascade Range volcanoes. In addition, its sequence of eruptive products records more changes in
chemical and mineral composition than is typical of the other volcanoes. These fluctuations reflect many differences
in physical or chemical conditions, or both, in the source magma or magmas.
Much of the known eruptive record of Mount St. Helens has been determined from
study of fragmental deposits, including study of flowage deposits (Crandell, 1987) and tephras described herein.
Fragmental deposits are particularly useful for study of eruptive histories because they form series of strata
that are highly susceptible to erosion, which exposes their stratigraphic sequences. They are also widespread,
and they commonly char, bury, and preserve carbonaceous material, which provides radiocarbon ages for the eruptive
events.
Even the complex eruptive history recognized for Mount St. Helens does not
include all of its past eruptive events. For example, several indistinct, poorly preserved strata of unknown origin
lie between tephra sets C and M; they suggest additional, unidentified eruptions. Moreover, comparison of small
observed eruptions in October 1980 with the resulting obscure deposits indicates that comparable eruptions in the
past might not have been detected during this study of tephra.
The youth of Mount St. Helens is demonstrated by the recency of both its oldest
deposits and its visible cone. The oldest known deposits are only about 40,000 or perhaps 50,000 years old, in
contrast to rocks at other major Cascade Range volcanoes that are more than a hundred thousand years old. The cone
of Mount St. Helens is also young compared to other large Cascade Range volcanoes. Before 1980, Mount St. Helens
was a relatively symmetrical, little-eroded structure emplaced over older ridges and valleys (fig.
1). Its symmetry and smooth slopes were recognized by early explorers and scientists alike as evidence that
the edifice was young. Just how young was surprising; most rock formations visible on the cone before 1980 were
less than 2,500 years old, and those on the upper part were less than 1,000 years old.
Mount St. Helens has also been much more active, especially explosively active,
during the last 40,000 years than its sister Cascade Range volcanoes. During the past 4,000 years, for example,
it produced more than 50 identifiable tephra strata, numerous pyroclastic-flow and hot lahar deposits, several
domes, and lava flows that flowed down all sides of the volcano. In contrast, during that same 4,000-year period
Mount Rainier produced only a few tephra deposits (Mullineaux, 1974) and relatively few deposits of pyroclastic
flows, hot lahars, or lava flows (Crandell, 1971).
Subdivisions of eruptive history
The eruptive history of Mount St. Helens can be subdivided into (1) old
and modern segments according to major compositional changes and (2) several stages and periods according to the
episodic nature of its eruptions (table 7).

Table 7. Classification of pre-1980 eruptive history, Mount St. Helens.
(12K)
|
Old Mount St. Helens
Compositionally, an early silicic volcano that existed until about 2,500
years ago can be distinguished from the modern, more mafic cone. The early volcano, termed "old Mount St.
Helens" by Verhoogen (1937), consisted of dacite and silicic andesite. It produced abundant tephra, pyroclastic-flow
deposits, domes, and short lava flows, some of which in turn generated other pyroclastic flows and lahars. Close
to the volcano, pumiceous tephra deposits of old Mount St. Helens commonly are as thick as several meters (fig.
3). Pyroclastic-flow deposits from the old volcano are major constituents of thick, flat-topped stacks of strata
that partly fill valleys leading away from the cone (Crandell, 1987). Although remnants of old domes and lava flows
are sparse, lithic pyroclastic-flow deposits provide evidence of their existence (Crandell, 1987). The dominantly
fragmental deposits of the old volcano include the rocks of the "old Mount St. Helens series" of Verhoogen
(1937, p. 268) and the "ancestral volcanic center" of Hopson (1971).
Modern Mount St. Helens
The modern volcano began with the eruption of mafic magma shortly after
2,500 years ago. Most eruptions of the modern volcano occurred during one of two main episodes: between 2,500 and
l,500 years ago, and between about 500 years ago and the present. A brief eruptive episode occurred about 1,200
years ago.
The mafic eruptions that initiated the modern volcano produced andesitic and
basaltic tephra, pyroclastic flows, and hot lahars as well as lava flows; however, not all magma erupted by the
modern volcano was mafic. Even after the mafic magma appeared, dacite continued to be erupted intermittently, producing
tephra, pyroclastic flows, and domes. The resulting modern cone is a complex pile of mafic lava flows, dacitic
domes, and dacitic and andesitic deposits of pyroclastic flows, lahars, and tephra, all of which have been intruded
by dikes and plugs of basalt, andesite, and dacite.
Eruptive Stages and Periods
Both the old and modern volcanoes were strongly episodic as well as variable
in composition, and thus the eruptive history can be divided into many parts. The pre-1980 history of the volcano
as now recognized includes four eruptive stages, the Ape Canyon, Cougar, Swift Creek, and Spirit Lake; the Spirit
Lake is further divided into six eruptive periods (Crandell, 1987) (table 7). Each stage
and each period represents an episode of multiple eruptions characterized by close association in time, similarity
in composition, or both.
Initially, Mount St. Helens' eruptive record was subdivided into nine eruptive
periods (Crandell and others, 1981; Mullineaux and Crandell, 1981). In that classification, eruptive periods before
4,000 years ago had durations of thousands of years, in contrast to younger ones that had durations of only centuries.
To remedy that disparity, Crandell (1987, p. 12-13) reclassified the first three periods as stages, and combined
the last six periods into a single stage. In the revised classification, each stage includes repeated episodes
of activity and intervening dormancy spread over more than a thousand years.
Deposits of the first two stages, Ape Canyon and Cougar, are relatively poorly
preserved, and we have only sketchy knowledge of their eruptive history. All deposits of these stages were subjected
to severe erosion and other disturbances during the last major glaciation and have been weathered for a long time
compared with other Mount St. Helens products. Deposits of the following Swift Creek stage are much better preserved;
however, they have been subjected to rigorous processes caused by a cold, late-glacial climate and more than 10,000
years of postglacial weathering. In contrast, products of the youngest (Spirit Lake) stage are remarkably well
preserved, and the eruptive history of that stage is much better known.
Each stage was separated from the next by a long dormant, or at least relatively
quiet, interval that can be inferred from buried weathering profiles or absence of eruptive products. Some evidence
suggests that at least minor eruptions did occur during intervals between the first three stages, whereas no evidence
has been seen that suggests any such activity between the latest two stages, the Swift Creek and Spirit Lake.
Ape Canyon Stage
The Ape Canyon stage began with the small-volume eruptions that apparently
record the birth of the volcano. The first evidence known of a Mount St. Helens is in the multiple, thin beds of
layer Cb, which record small, mild to moderately explosive eruptions. These eruptions probably also created domes
and perhaps pyroclastic flows, but tephra of layer Cb is their only identified product. The outbursts that produced
layer Cb may have occurred in rapid succession because no evidence of a pause long enough to form even an incipient
soil was found within the layer.
The volcano then was dormant long enough for an oxidation profile to form in
the upper part of layer Cb. The length of time represented by the profile is not known, but comparison with profiles
in younger, better dated deposits suggests more than a thousand years. Evidence from studies of Mount St. Helens
tephras far downwind suggests the possibility that a much longer time, perhaps as much as 10,000 years, passed
between deposition of layer Cb and younger layers of set C (Busacca and others, 1992).
Small-volume eruptions of pumice also began the next, main series of Ape Canyon
events. These were followed, without evidence of a pause, by large-volume eruptions that produced layers Cw and
Cm. Those were followed in turn by repeated outbursts that produced thin beds of probable tephra and thicker deposits
of probable ash-cloud origin. That series of eruptions was interrupted at least three times by pauses long enough
for weakly oxidized soils to form. The Ape Canyon tephra eruptions then culminated in highly explosive outbursts
that produced the voluminous layers Cy and Cs. Layer Cs, which may be correlative with layer Cy, is the largest
volume tephra known of Pleistocene age.
During that main series of Ape Canyon events, the volcano also produced pyroclastic
flows and surges and their associated ash clouds as well as mudflows (Hyde, 1975; Crandell, 1987). Prismatically
jointed lithic blocks in one mudflow deposit suggest the presence of one or more domes (Crandell, 1987, p. 19).
The main series of Ape Canyon eruptions probably spanned at least 2,000 years.
Two radiocarbon ages from just underneath tephras erupted during that time (W-2661 and W2976, table
2) and one from a volcanic mudflow (Hyde, 1975) at Mount St. Helens are between 38,000 and 36,000 years B.P.
A radiocarbon age of about 33,650 years B.P. from 25 cm above tephra of Ape Canyon age in Nevada (Davis, 1978,
p. 45) is consistent with the ages obtained from deposits near the volcano.
Thick flowage deposits of the Ape Canyon stage extended down the North Fork
Toutle valley and probably aggraded it to at least as far downstream as the Cowlitz River valley (Crandell, 1987;
Scott, 1988). Those deposits must have also dammed the North Fork Toutle valley north of Mount St. Helens to produce
the first of many versions of Spirit Lake.
Ape Canyon-Cougar Interval
About 15,000 years passed between Ape Canyon and Cougar stage eruptions.
No unequivocal primary eruptive products have been identified at Mount St. Helens that represent that period, although
some evidence suggests that the volcano was not completely dormant. Fine, ash-rich detritus accumulated on large
areas of uplands during at least three separate episodes during this interval. In addition, thin, discontinuous
lenses of small pumice lapilli within those deposits suggest that some tephra was erupted; however, these pumice
deposits are not sufficiently voluminous or well preserved to be identified satisfactorily as to origin, and thus
unambiguous evidence of eruptions is lacking. That lack of evidence, however, could be due only to lack of preservation
because voluminous tephras may have been erupted and carried only northeasterly. In the Cascade Range to the northeast,
any such tephra would be severely eroded and not easily found. Farther to the northeast in Canada, two tephras
that are between 35,000 and 20,000 years old and are characterized by cummingtonite have been identified (Westgate
and Fulton, 1975).
Cougar Stage
The Cougar stage, which apparently lasted only 2,000-3,000 years, is characterized
by tephra eruptions that were less voluminous than those of Ape Canyon time but show more compositional variation.
Those eruptions also produced large pyroclastic flows and lahars (Crandell, 1987), one or more lava flows of dacite
or siliceous andesite (C.A. Hopson, written commun., 1974), and probably one or more dacite domes of similar composition.
Two episodes of tephra production are identified within the Cougar stage and are separated by enough time to form
an oxidized soil profile.
The Cougar stage apparently began with mildly explosive or nonexplosive events
that produced lahars, a debris avalanche, and a siliceous-andesite lava flow (Crandell, 1987, p. 24). Explosive
eruptions then created large pumiceous pyroclastic flows that travelled down the southeast, south, and west sides
of the volcano (Crandell, 1987); deposits of those flows are characterized by hypersthene and hornblende. These
eruptions were followed by outbursts that produced the multiple pumiceous layers of tephra set M and some coeval
ash deposits that probably were derived from pyroclastic flows. The first set M tephras are characterized by cummingtonite,
which is progressively replaced upward in the set by hypersthene.
Eruptions of now recognizable tephra layers stopped temporarily after deposition
of set M. Ashy fine material that accumulated on top of the set suggests, however, that at least minor eruptions,
perhaps of tephra or pyroclastic flows, continued.
The next eruptive episode of this stage produced a sequence of tephras very
different from earlier ones. Eruptions that produced tephra set K as recognized were small volume, intermittent,
and repetitive in terms of scale and composition. Some larger volume eruptions may have occurred at about that
time, as suggested by strata in an outcrop northeast of the volcano, but the age relation of these strata to set
K tephras is not known. Set K was followed by eruption of voluminous pyroclastic flows that moved down the south
and southeast flanks of the volcano. Those flows are the last known products of the Cougar stage.
During Cougar time, large pyroclastic flows and lahars filled the Lewis River
valley south of Mount St. Helens to a depth of more than a hundred meters and aggraded the valley far downvalley
(Hyde, 1975; Crandell, 1987). Detritus from the volcano so overwhelmed the Lewis River that pyroclastic-flow deposits
of Cougar age have surface gradients of as much as 25 m/km from north to south across the Lewis River valley directly
south of Mount St. Helens.
The ferromagnesian mineral suites in products of Cougar time record different
magmatic conditions at various times during this stage. Experiments of several investigators (see Geschwind and
Rutherford, 1992, and references therein) indicate that magmas characterized by cummingtonite last equilibrated
at lower temperature and perhaps higher water content than those characterized by hypersthene. Several differences
or changes between relatively cool and hot magmatic conditions are recorded in the Cougar-stage products. It is
not known, however, whether these changes represent different source magmas, different parts of a nonhomogeneous
magma, or changes in a magma with time.
The hypersthene-rich ferromagnesian suites of early products suggest that the
source magma had equilibrated at a higher temperature than that of last magma erupted at the end of the previous,
Ape Canyon, stage. But, by the time the first layers of set M were erupted, the dominance of cummingtonite suggests
that the source magma or magma batch for the set M tephras had equilibrated at a lower temperature. No intervening
products characterized by both cummingtonite and hypersthene are known.
During set M eruptions, temperatures of the source magma apparently became
progressively higher, as indicated by hypersthene substitution for cummingtonite upward in the sequence. Such a
change could have resulted from progressive heating of the source magma by injection of new magma or perhaps by
tapping of successively deeper parts of a magma body (Hopson and Melson, 1990). Presence of olivine in layer Mo
suggests that a new magma, if present, was mafic.
The sequence of set K tephras and the pyroclastic-flow deposits that followed
set M indicates again a relatively low-temperature source magma and a return to a higher temperature magma.
Cougar-Swift Creek Interval
Little is known about the relatively quiet interval of about 5,000 years
that followed the Cougar stage. Accumulation of ash-rich fine sediments on uplands suggests some volcanic activity,
but no deposits containing pumice lapilli were seen in those sediments. Because this interval occurred during the
latter part of the last major glaciation, eruptive products of the time could have been so severely eroded or altered
that they were not identified in this study.
Swift Creek Stage
During the Swift Creek stage, between about 13,000 and 10,500 years ago,
the volcano produced large volumes of pyroclastic flows and moderate to large volumes of tephras that extend hundreds
of kilometers downwind. The Swift Creek stage includes two distinct episodes of tephra production, one about 13,000
years ago and the second between about 12,000 and 10,500 years ago. Between these episodes, multiple pyroclastic
flows and lahars built extensive valley fills (Crandell, 1987).
The Swift Creek stage began with the production of tephras and ash-cloud deposits,
and ash-cloud deposits continued to be produced throughout the time of deposition of set S tephras. The final two
eruptions of set S produced the two largest volume tephras since Ape Canyon time. Although only the last two tephras
of set S were of such large volume, as many as three beds of set S have been recognized at multiple sites in eastern
Washington as far as 300 km east of the volcano (Foley, 1976, 1982; Hammatt, 1976; Moody, 1977; Mullineaux and
others, 1978; Busacca and others, 1992). The presence of ash-cloud deposits interbedded with tephra of all parts
of set S indicates that pyroclastic flows were produced repeatedly during that part of the Swift Creek stage.
Set S tephra was followed by many lahars and lithic pyroclastic flows; some
lithic pyroclastic flows probably were derived from domes (Crandell, 1987). Flowage deposits were voluminous enough
to produce extensive valley fills, especially southeast of the volcano (Crandell, 1987, p. 36). As the pyroclastic
flows and lahars filled valleys, ash-rich deposits accumulated on upland surfaces.
The next Swift Creek events produced thin ash deposits at the base of set J.
The volcano then erupted at least three large-volume tephras but apparently no associated pyroclastic flows. Ash
from these highly explosive outbursts extended far to the east of the volcano. The resulting ash beds have been
identified not only in eastern Washington but also as far as Montana (Carrara and others, 1986).
The final eruptions of Swift Creek time produced the thick, coarse tephra layer
Jg, which extends toward the west. Limited distribution relative to thickness and grain size suggests that either
the eruptive column was low or the winds were of low velocity during its eruption.
As in the Cougar stage, the ferromagnesian minerals in Swift Creek deposits
record differences in source-magmas conditions from one eruptive episode to another. The cummingtonite in set S
suggests a magma that had equilibrated at a lower temperature than the source magma of the last-known eruptions
of Cougar time. The disappearance of cummingtonite and its replacement by hypersthene in tephras of set J indicate
again a relatively high temperature in the source magma. The last Swift Creek tephra erupted is also the least
silicic and may record mixture of invading mafic magma and preexisting dacitic magma.
Swift Creek-Spirit Lake Interval
During this interval, from about 10,500 to 4,000 years ago, Mount St. Helens
apparently was completely dormant. No evidence has been found that indicates that the volcano erupted at all during
that time; a search during many years has turned up no evidence of eruptive products attributable to that interval.
Nor, in contrast to the earlier intervals between stages, is there any oxidized, ash-rich bed that would suggest
unrecognized events during this interval. Obviously, the possibility of minor eruptions cannot be ruled out because
their products could have filtered down into the disturbed soil zone at the top of the deposits of Swift Creek
age. This interval, however, is the longest in the history of the volcano for which we have no evidence whatever
of eruptive activity.
Spirit Lake Stage
Eruptions during the Spirit Lake stage were responsible for building the
volcano generally recognized as Mount St. Helens. The six eruptive periods of the Spirit Lake stage produced the
rocks that make up the visible cone and record the compositional change from the older Mount St. Helens to the
more mafic and variable modern volcano. Highly explosive eruptions of voluminous, pumiceous tephra are notable
features of the earliest period. During later periods, domes, pyroclastic flows, and lava flows became more important.
Smith Creek Period
The first period of Spirit Lake time consists of three eruptive episodes
that differ distinctly in character. The Smith Creek period began with several explosive pulses of small to moderate
volume that produced the thin but widespread pumiceous layer Yb. The distribution of these beds shows that wind
directions changed between or during the eruptions. Deposition of layer Yb was followed, with no apparent pause,
by numerous but probably less gas rich and less vigorous ejections of lithic to somewhat vesicular tephras of layer
Yd.
Mount St. Helens then was dormant for a few hundred years. During that time,
surficial processes formed a thin, incipient soil that is commonly topped by sparse carbonaceous material. This
soil is weak but persistent and can be identified from northeast to southeast of the volcano.
Highly explosive and voluminous eruptions characterize the second phase of
Smith Creek time. The second phase started with the discharge of layer Yn, the largest volume tephra of Holocene
time. Layer Yn is present along a narrow lobe to the north-northeast that extends for at least several hundred
kilometers across the northwestern United States and into Canada (Crandell and others, 1962; Westgate and others,
1970). The tephra eruption was closely followed by one or more pyroclastic flows that produced thick ash-cloud
deposits; these deposits directly overlie layer Yn close to the volcano. Another highly explosive and voluminous
pumice eruption then produced the Ye tephra, which was carried eastward across Washington State and at least as
far as Idaho (Smith and others, 1977). Eruption of the Ye tephra was also followed by pyroclastic flows, which
produced ash-cloud deposits that cover the tephra layer near the east base of the volcano.
Tephra eruptions that followed layer Ye were much smaller and are characterized
by less vesicular pumice and higher proportions of lithic fragments. Interbedding of ash-cloud deposits and abundance
of lithic clasts suggest that the eruption of tephra was interspersed with formation of pyroclastic flows and domes.
This activity apparently continued with no pauses long enough to form recognizable soils until the end of the Smith
Creek period. One conspicuous, hot lithic pyroclastic flow of late Smith Creek time entered the Smith Creek valley,
picking up and charring logs (Crandell, 1987); its associated ash cloud spread over adjacent slopes and formed
the charcoal-bearing ash bed ya.
Pyroclastic-flow and lahar deposits of the Smith Creek period raised the level
of the fan on the north flank of the volcano, and lahars extended down the North Fork Toutle River Valley at least
50 km below Spirit Lake. The thick deposits of Smith Creek age that crop out downvalley indicate that an early
Spirit Lake was formed or expanded at that time.
Radiocarbon dates show that the Smith Creek period began shortly after 4,000
years ago and ended at some time after 3,350 years ago. Ages at the base of layer Yn of about 3,500 years demonstrate
that the dormant time between eruption of layers Yb and Yd lasted no more than a few hundred years.
Smith Creek-Pine Creek Interval
This interval lasted at most about 300 years and may have been much shorter.
Some eruptions occurred during the interval, but apparently none were strong enough to produce lapilli-size clasts
on the lower flanks of the volcano or nearby. Only the ash bed pbp, with its faint bedding, records activity during
the Smith Creek-Pine Creek interval. The thin lamina within ash bed pbp exhibit successive changes in ferromagnesian
mineral composition that record a series of some kind of small-volume eruptive events. They also apparently record
a progressive change from a relatively low temperature magma, characterized by cummingtonite, to a higher temperature
magma characterized by hypersthene. The magma under Mount St. Helens at the time may have been heated by injection
of mafic magma that did not reach the surface until several hundred years later, during the eruptions of Castle
Creek time.
Pine Creek Period
Pine Creek time, relative to Smith Creek time, was marked by much lower
explosivity. It was characterized by discharge of small-volume tephras and large-volume pumiceous and lithic pyroclastic
flows (Crandell, 1987). Lithic pyroclastic-flow deposits, probably derived from relatively nonexplosive eruptions
of dacite domes, extend as far as 18 km from the volcano (Crandell, 1987).
The earliest eruptions of the Pine Creek period probably formed a dacite dome
or cryptodome. The first tephra eruption, of layer Pm, was strongly explosive but not voluminous. The tephra contains
abundant fresh, lithic fragments of hypersthene-hornblende dacite. Evidently, degassed magma or rock of Pine Creek
composition was emplaced on or near the surface before being fragmented during the eruption of layer Pm. Several
successive small-volume tephra eruptions produced the thin, multiple beds that make up layer Ps; these beds contain
abundant lithic clasts as well as clasts of pumice. The eruptions of layers Pm and Ps presumably were interspersed
with pyroclastic flows and domes that provided a source of fresh, lithic clasts.
Although no recognized tephra deposits are present between layers Ps and Pu,
ash of probable ash-cloud origin suggests that pyroclastic flows formed during that time.
When tephra eruptions resumed, they produced the thin, multiple beds of layer
Pu, which are remarkably similar to those of layer Ps. As during the early part of Pine Creek time, abundance of
fresh lithic clasts indicates that domes or cryptodomes formed between tephra eruptions.
The final tephra of Pine Creek time records at least three explosive events,
during which gas-rich magma was erupted along with lithic fragments. Yellow-gray ash of probable ash-cloud origin
that is associated with beds of layer Py indicates that at least one pyroclastic flow occurred during the eruptions
of tephra.
Pine Creek time spanned most of an interval from about 3,000 to 2,500 years
ago. During that time, lahars and fluvial deposits consisting mostly of newly erupted material strongly aggraded
the valley floors of the North Fork Toutle River and other rivers that headed on the volcano. They raised the surface
of the fan that impounds Spirit Lake and, 40 km down the North Fork Toutle valley, blocked a small tributary valley
to create the basin that holds Silver Lake (Mullineaux and Crandell, 1962). They also formed a continuous fill
across the floor of the Cowlitz River Valley near Castle Rock and another fill in the Lewis River valley, which,
near Woodland, was about 7.5 m higher than the present floodplain (Crandell and Mullineaux, 1973).
Pine Creek-Castle Creek Interval
This interval was short, lasting at most about 300 years and very likely
much less. Evidence of volcanic activity is limited to that provided by ash bed v, which suggests that some volcanic
events of small volume occurred. The lack of charred vegetation in or at the top of ash bed v suggests that no
dense stand of vegetation was established during this interval.
Castle Creek Period
The defining feature of this period is the first appearance of mafic magma
at the surface, which initiated the modern Mount St. Helens and its variety of rock composition. Andesite, dacite,
and basalt all were erupted during Castle Creek time, producing pyroclastic flows, surges, and tephra as well as
lava flows. Volumes of explosively erupted deposits were moderate or small.
The first known eruptive products of the period are andesitic and are characterized
by ferromagnesian suites of hypersthene and augite. The early Castle Creek eruptions resulted in at least one lava
flow and created lahars (Crandell, 1987). Next, explosive eruptions produced the tephra layer Bh. Similar explosions
that followed resulted in layer Bo, in which olivine dominated ferromagnesian suites for the first time in Mount
St. Helens history. Repeated explosions created several different shower beds within layer Bo, as well as pyroclastic
flows and hot lahars. Intercalation of tephras with pyroclastic-flow and lahar deposits suggests frequent changes
in eruptive behavior, which probably included initial fountaining of tephra during eruptions that later produced
pyroclastic flows, lahars, and lava flows.
After layer Bo, milder eruptions of undetermined character produced mafic ash,
followed by ash that was more silicic. These deposits, which formed ash bed ba, probably include detritus from
both small tephra eruptions and from ash clouds derived from pyroclastic flows. As ash bed ba accumulated, the
small-volume, slightly vesicular dacitic tephra layer Bd was erupted. Dacitic pyroclastic flows of the same composition
as layer Bd provide radiocarbon dates of 2,200-2,000 years for those deposits (Crandell, 1987). After a pause of
200-400 years, another explosive eruption produced the dacitic tephra layer Bi, which is highly vesicular and has
a ferromagnesian mineral suite slightly different from that of layer Bd. Radiocarbon ages of approximately 1,800
years, obtained from both below and above layer Bi, indicate that eruption of layer Bi was distinctly later than
that of layer Bd.
After formation of layer Bi, only olivine basalt is recorded by the tephra
sequence; the basaltic scoria of layer Bu records at least two mildly explosive events. At one site, however, an
andesite lava flow overlies dacitic pyroclastic-flow deposits and underlies a basalt lava flow (Crandell, 1987,
p. 57-59). Thus, at least one andesitic eruption occurred between the eruptions of dacite and basalt.
Basaltic lava flows as well as tephra were produced at the end of Castle Creek
time. Multiple thin flow units of basalt overlie at least part of layer Bu on the north flank of the volcano; the
lava flows were emitted after at least the first eruption of layer Bu. Fountaining that produced tephra may have
been followed by quieter emission of the lava flows.
The largest basalt flows of late Castle Creek time, however, are pahoehoe flows
on the south flank of Mount St. Helens. There is no physical overlap of the basalt flows on the north with the
pahoehoe flows on the south, and radiocarbon dates do not firmly determine which is younger (Crandell, 1987). Consequently,
the age relation of the pahoehoe flows and other basalts of Castle Creek time is uncertain.
Castle Creek eruptions followed those of Pine Creek time after only a short
period and continued for almost 1,000 years.
Sugar Bowl Period
The Sugar Bowl episode was short and markedly different from other periods
in Mount St. Helens history. It produced the only unequivocal laterally directed blast known from Mount St. Helens
before the 1980 eruptions (Crandell and Mullineaux, 1978).
During Sugar Bowl time, the volcano first erupted quietly to produce a dome,
then erupted violently at least twice producing a small volume of tephra, directed-blast deposits, pyroclastic
flows, and lahars (Crandell and Hoblitt, 1986; Crandell, 1987). The Sugar Bowl blasts, described initially as only
one (Crandell and Mullineaux, 1978), were much smaller than the one in 1980, extending only about one-third as
far as that of 1980. In composition, fragmental deposits of the Sugar Bowl period are similar to rock of Sugar
Bowl dome.
Radiocarbon analyses and stratigraphic relations indicate that the Sugar Bowl
eruptions occurred about 1,200 years ago, about midway between the Castle Creek and Kalama periods. The morphogically
similar East dome, in contrast, has no fragmental deposits associated with it, and its age is not closely known.
Absence of set B tephras on East dome indicate that it was emplaced after the Castle Creek period, and presence
of set W tephras on it show that it existed before Kalama time. It could have risen at any time between those two
periods, and whether or not it is coeval with the Sugar Bowl dome is not known.
Except for the dome itself, Sugar Bowl eruptions produced only deposits of
small volume and probably occurred within a short time, perhaps a few years. Sugar Bowl eruptions caused no major
changes in the shape or size of the volcano but did form a prominent dome on the north flank of Mount St. Helens.
Kalama Period
In A.D. 1480, or possibly late in A.D. 1479, a highly explosive pumice eruption
opened the Kalama period with discharge of tephra layer Wn. The Kalama period continued for perhaps 300 years and
comprise a wide variety of magma compositions and kinds of eruptions. It included highly explosive ejection of
voluminous dacitic and andesitic tephras and pyroclastic flows, dacitic pyroclastic flows, nonexplosive eruption
of andesite lava flows, and rise of the dacitic summit dome.
Eruption of layer Wn produced the most extensive and most voluminous tephra
of Kalama time; layer Wn has been identified as far as 500 km northeast of Mount St. Helens (Smith and others,
1977). Reworking of that pumice layer, where thick, produced fluvial and laharic deposits locally at the northeast
and south bases of the volcano (Crandell, 1987). A dome probably formed next, before eruption of the relatively
small tephra layer Wa. The dome provided layer Wa with abundant fresh lithic fragments of the same mineral composition
as the new magma. The presence of more than one bed or zone in both layers Wa and Wb, along with abundant fresh
lithic clasts, indicates that multiple small explosive pulses and dome formation occurred between A.D. 1480 and
A.D. 1482, when the next voluminous and highly explosive eruption produced layer We. One or more pyroclastic flows
probably were created during that event because ash probably derived from pyroclastic flows is intermingled with
and overlies the upper part of the layer. Layer We also extended far downwind and has been identified eastward
more than 300 km from Mount St. Helens (Smith and others, 1977).
Perhaps about the time of eruption of layer We, pyroclastic flows of pumiceous
and lithic dacite began to move down the southwest flank of the volcano (Hoblitt and others, 1980). The magma erupted
gradually became more mafic. Explosive, dacitic eruptions were followed by weaker eruptions of andesitic tephra
and the quiet emission of andesitic lava flows, which mantled much of the south flank of the volcano. Some beds
of tephra set X probably were ejected during early phases of eruptions that produced lava flows.
The andesitic eruptions were followed by rise of a large dome, perhaps starting
during the first half of the 17th century (Hoblitt, 1989); that dome made up the summit of Mount St. Helens until
early 1980. Avalanches of hot debris from the dome swept down all sides of the volcano, formed pyroclastic flows
and lahars, and covered the upper parts of many Kalama lava flows (Hoblitt and others, 1980; Crandell, 1987). The
rounded summit dome and smooth profiles of the avalanches and lava flows were primarily responsible for the smooth,
youthful appearing cone of the pre-1980 Mount St. Helens.
The principal growth of the volcano during the Kalama period probably occurred
within about 200 years, but debris avalanches from the dome continued for about another 100 years (Hoblitt, 1989;
Pallister and others, 1992).
Changes in composition during Kalama time were gradual but distinctive, from
dacite to andesite and back to dacite. Patterns of trace elements along with other compositional changes indicate
that the changes resulted from injection of mafic magma into a preexisting dacite (Hoblitt, 1989; Pallister and
others, 1992)
Goat Rocks Period
The Goat Rocks eruptive episode was short and produced relatively small
volumes of magma. Its first eruptive event was a single, highly explosive, moderate-volume eruption in A.D. 1800
that produced the pumiceous, dacitic layer T. That layer spread along a narrow path northeastward across Washington,
Idaho, and into Montana (Okazaki and others, 1972, p. 81). The tephra eruption apparently was not accompanied by
other events but was followed later in the same year (Yamaguchi and others, 1990) by the andesitic lava flow called
the "floating island" flow by Lawrence (1941).
No eruptions have been documented for the next three decades, but minor ones
were reported from A.D. 1831 to A.D. 1857 (Holmes, 1955). In A.D. 1842, the largest volume of those eruptions dumped
a reported half-inch of ash on The Dalles, Oregon. Goat Rocks dome was extruded at about that same time (Crandell,
1987, p. 88). The dome probably was constructed incrementally through several years during the 1840's, during which
time many eruptions were observed (Holmes, 1955). Growth of the dome caused dome-rock avalanches, pyroclastic flows,
and lahars; the flowage deposits created a broad fan below the dome on the north flank of the volcano (Hoblitt
and others, 1980, p. 558; Crandell, 1987). The last eruption usually attributed to the Goat Rocks period occurred
in 1857, although a study of old records suggests that minor eruptions of Mount St. Helens might have occurred
in 1898, 1903, and 1921 (Majors, 1980, p. 36-41).
The relatively small volume eruptions of Goat Rocks time produced no major
changes in the size or shape of the volcano.
HAZARDS FROM TEPHRA ERUPTIONS
Future eruptions of Mount St. Helens are certain, and some of those eruptions
will endanger lives and property. Tephra generally is less of a threat to lives than flowage events such as pyroclastic
flows and lahars. Nevertheless, tephra can cover such large areas that it can cause even greater overall loss to
people and property than flowage events.
Although future eruptions certainly will occur, their specific character and
timing cannot be predicted. Only generalized forecasts can be made, based on patterns of past activity, and even
those are necessarily uncertain. Probabilities of various kinds and magnitudes of events can be estimated, however,
from the volcano's eruptive record, and hazard maps based on these probabilities can be drawn to guide response
planning. And, although the timing of future eruptions cannot be predicted, seismic monitoring would be expected
to detect movement of large amounts of magma at Mount St. Helens, which in turn would provide warning before another
large eruption occurs (Pallister and others, 1992).
Tephra causes physical, medical, psychological, and social problems, especially
close to the volcano. The severity of most effects decreases rapidly with increasing distance because of decreasing
thickness of tephra deposited, yet the overall damage beyond the immediate vicinity of the volcano can be great
because of the vast areas affected.
Tephra endangers people and property chiefly by impact and heat of large fragments,
burial and load from thick accumulations, ash inhalation, and many other effects resulting from dispersion of ash
in air and water (see Wilcox, 1959b, Blong, 1984, and Scott, 1989, for more detailed descriptions). Close to a
volcano, the chief dangers are large fragments and thick ash. Most deaths from tephra eruptions are caused by fragment
impact or collapse of roofs caused by the weight of tephra. Large fragments can also kill or injure people and
animals by heat, penetrate and otherwise damage structures, and start fires. In addition to crushing roofs, thick
ash deposits commonly disable power and communication lines, block transportation routes, and bury crops. People
have been killed or injured by inhaling large amounts of ash, especially if it is hot, and injury to eyes and respiratory
systems can be serious for both people and animals. Ash also commonly overloads water supply and sewer systems,
and development of a cemented crust on ash deposits can lead to excessive runoff that in turn causes secondary
mudflows.
At great distances, where tephra is fine grained and dispersed, it can still
cause serious injuries to eyes and respiratory systems. The thin deposits can also severely hamper transportation
and communication facilities. Even at hundreds of kilometers from a volcano, ash suspended in the air can limit
transportation by causing darkness during daylight hours and otherwise reducing visibility and by damaging engines
and other equipment. Growing crops can be set back or destroyed by thin ash, but future crops typically benefit
from the addition to the soil.
Recent events make it clear that ash is extremely dangerous to jet aircraft,
even at great distances from a source volcano. On several occasions, highly dispersed ash has damaged jet engines
sufficiently to cause temporary failure and accompanying loss of altitude and risk of a crash. In most of these
encounters, ash particles have also caused extensive damage to other parts of the aircraft (Casadevall, 1991).
Medical, psychological, and social effects (see Blong, 1984) are commonly long
term as well as immediate. Depression, anxiety, and stress were high in areas affected by tephra in the first two
years after the 1980 Mount St. Helens eruptions (Shore and others, 1987), and ensuing political disruption, anger,
and litigation continued for many more years.
Fortunately, losses from tephra effects can be greatly minimized, except for
those from large-volume eruptions and near the volcano. In some cases, the fallout can simply be avoided, and in
many others protective measures can limit adverse effects. Avoidance is feasible except close to the volcano primarily
because of the time wind-carried tephra takes to reach any given location after its eruption has begun. That time
can allow people to move out of the path of the tephra; obviously such a response is increasingly feasible with
increasing distance from the volcano. Avoidance by evacuation before an eruption begins, however, generally is
not appropriate. Neither the character nor timing of such an eruption could be specifically predicted, a large
number of people might have to be moved, and the area predicted to be at risk would change with each anticipated
change in wind direction.
In contrast, protective measures are relatively straightforward, and many are
easy to implement. Taking shelter in sturdy structures, and using masks or covers to protect people and equipment
from ash, can significantly reduce damage. For best results, mitigative measures should be both planned well in
advance and easy to implement on short notice. Obviously, early notification that tephra has been erupted and information
about its direction and speed are critical in order for protective measures to be taken before the tephra arrives.
In practice, effective reponse to eruptions, even when the hazards have been
assessed and described, has been difficult (see Peterson, 1988, and Peterson and Tilling, 1993, and references
therein). An important factor is that predictions (and thus warnings) of future eruptions must be probabilistic;
they are not specific as to either time or character of eruptions. Sorenson and Mileti (1987) pointed out that
warnings need to be not only specific, but also certain, consistent, accurate, and clear. In the absence of predictions
that are specific and certain, response plans must be drawn to cover many possible times and situations, perhaps
too many to be readily understood and accepted. Those same uncertainties make it difficult to maintain a high state
of readiness.
To date, volcanologists' mitigation efforts have consisted chiefly of hazards
assessments and monitoring to predict eruptions. Recently, the importance of effective communication of that information
has been emphasized (Peterson, 1988). Yet another important factor is that of detection of hazardous events when
they actually occur so that civil officials and the general public can be warned. Such warnings are more desirable
than conditional warnings of "possible" or "probable" events, which usually are all that can
be provided in advance. Warnings of real events in progress meet the criteria of being specific and certain (Sorenson
and Mileti, 1987) and enable civil officials and the public to react with greater confidence.
The risks posed by tephra are particularly appropriate for mitigation by warnings
based on detection of events already in progress. In many cases, erupted tephra can be detected and tracked visually
from the ground or air, and satellites can provide primary or supplemental information. Another method developed
recently (Hoblitt, R.P., written commun., 1992) tracks the downwind progress of erupted tephra by detecting the
lightning strikes that it causes.
Thus, tephra can be dangerous, but mitigation can be relatively successful
because of its characteristics. Success, however, requires adequate advance planning, detection of hazardous events
when they actually occur, and the capability of prompt response to those events.