Hydrogeologic Framework of the Big Lost River Basin, South-Central Idaho
Links
- Document: Report (8.8 MB pdf) , XML
- Larger Work: This publication is Chapter A of Characterization of water resources in the Big Lost River Basin, south-central Idaho
- Appendix: Appendix 1 (1.4 MB pdf)
- Data Release: USGS data release — Hydrogeologic framework of the Big Lost River Basin, south-central Idaho—Hydrogeologic framework model and well data
- Version History: Version History (1 KB txt)
- Download citation as: RIS | Dublin Core
Acknowledgments
B. Twining (U.S. Geological Survey) contributed the geophysical methods description and prepared the geophysical logs, and J. Paustian (U.S. Geological Survey) assisted with well-driller report data entry. The following individuals provided helpful technical insights, discussion, and suggestions during the preparation of this hydrogeologic framework: D. Hoekema, D. Owsley, W. Parham, A. Steimke, J. Sukow, and C. Tesch (Idaho Department of Water Resources); J. Bartolino, K. Blasch, N. Herrera, and S. Hundt (U.S. Geological Survey); A. Clark (Idaho Geological Survey); and A. Zinsser (geologist).
Preface
This is the first of three reports in a multichapter volume characterizing water resources in the Big Lost River Basin. These reports document the findings of a hydrogeologic investigation of the Big Lost River Basin that was jointly conducted by the U.S. Geological Survey, Idaho Department of Water Resources, and the Idaho Geological Survey from 2018 through 2021. Chapter A (this report) describes the hydrogeologic framework of the Big Lost River Basin. It includes a conceptual definition of the hydrogeologic units, a three-dimensional hydrogeologic-framework model, and a description of groundwater occurrence and movement. Chapter B (Dudunake and Zinsser, 2021) describes streamflow gains from and losses to groundwater in the Big Lost River between Mackay Reservoir and south of Arco, Idaho. Streamflow gains and losses were estimated from a series of four surface-water measurement events during pre- and post-irrigation season conditions from 2019 to 2021. Chapter C (Clark, 2022) describes groundwater budgets for the Big Lost River Basin from 2000 to 2019. The groundwater budgets will provide annual estimates for aquifer inflows and outflows. Collectively, these reports present a characterization of water resources in the Big Lost River Basin that will help address current challenges in water-resources management.
Abstract
Surface-water and groundwater resources in the Big Lost River Basin of south-central Idaho are extensively interconnected; this interchange affects and is affected by water-resource management for irrigated agriculture and other uses in the basin. Concerns from water users regarding declining groundwater levels, declining streamflows, and drought helped motivate an updated evaluation of water resources in the Big Lost River Basin. The hydrogeologic framework presented in this report provides a conceptual basis for understanding groundwater resources in the Big Lost River Basin and comprises three major parts: (1) conceptual description of four hydrogeologic units, (2) development of a three-dimensional hydrogeologic framework model representing the spatial distribution of the hydrogeologic units, and (3) a description of groundwater occurrence and movement. This hydrogeologic framework represents the first of three planned reports describing water resources in the Big Lost River Basin; subsequent reports are intended to present a groundwater budget for the basin and to describe the results of a series of events measuring gains to and losses from streamflow in the Big Lost River. This report was prepared by the U.S. Geological Survey in cooperation with the Idaho Department of Water Resources.
The Big Lost River Basin has four hydrogeologic units. First, the most heavily used hydrogeologic unit is the Quaternary unconsolidated sediments, which are further subdivided by grain size or other distinguishing characteristics into six subunits (boulders, gravel, sand, clay, cemented sediments, and soil), and which comprise the alluvial aquifer. Second, Paleozoic sedimentary rocks, primarily carbonates with some siliciclastic rocks, represent the major bedrock aquifer and contribute subsurface recharge at the margins of the alluvial aquifer. Third, Tertiary volcanic rocks, primarily andesite and dacite with lesser tuff, are locally important to water production, particularly in faulted and fractured zones. Fourth, Quaternary basalt rocks contain at least three water-bearing zones at the southern end of the Big Lost River Valley.
The three-dimensional hydrogeologic framework model describes the spatial distribution and general thicknesses of the hydrogeologic units and subunits and yields new insights into controls on water movement in the basin. The Quaternary unconsolidated sediments hydrogeologic unit that comprises the basin-fill alluvial aquifer is highly spatially heterogeneous. The Paleozoic sedimentary rocks hydrogeologic unit occurs at the valley margins and underlies tributaries throughout the basin, whereas the Tertiary volcanic rocks hydrogeologic unit primarily occurs in uplands in the western half of the basin. The Quaternary basalt hydrogeologic unit consists of multiple basalt flows that are interbedded with the Quaternary unconsolidated sediments unit in the southern end of the Big Lost River Valley. Subunits within the Quaternary unconsolidated sediments unit generally are coarse (sands and gravels) and well-sorted with high hydraulic conductivity along the central axis of the Big Lost River Valley. Quaternary unconsolidated sediments subunits at the margins of the valley tend to be more vertically discontinuous and contain more clay, which creates locally confining layers in some areas. A laterally continuous clay subunit is present about 50 to 100 feet below the land surface around the town of Arco, Idaho, and causes confining conditions in some areas.
Groundwater flow generally mimics topography although wide spacing between long-term groundwater-level monitoring wells limits detailed interpretation. The alluvial aquifer is most heavily used (primarily by irrigation and domestic pumping) within 250 feet of the land surface although deeper bedrock wells occur at the valley margins and in the basalt flows around Arco. Potentiometric-surface altitudes vary substantially interannually and seasonally between Darlington, Idaho, and Arco; annual precipitation, irrigation demand, and recharge can result in raising or lowering of potentiometric surface altitudes over the irrigation season and likely affect streamflow in the Big Lost River. Historical surface-water losses occur in areas where the valley widens and substrates coarsen; historical surface-water gains occur in areas where the valley narrows, streamflow and irrigation recharge the alluvial aquifer, and confining layers occur in the subsurface. Vertical hydraulic connectivity between multiple water-bearing zones in the southern end of the Big Lost River Valley is unclear but continued monitoring of wells at multiple depths and additional aquifer tests could help improve understanding of hydraulic connectivity in this area.
In summary, this hydrogeologic framework provides an updated conceptual model for groundwater resources and a three-dimensional, data-derived hydrogeologic framework model used as a tool to represent the hydrogeologic units and subunits in the basin. The model shows the spatial distribution of hydrogeologic units and subunits and, combined with groundwater and surface-water data, illustrates their effect on groundwater movement and surface-water/groundwater interactions throughout the basin. Insights gained from this updated hydrogeologic framework will help inform current water-resource management in the Big Lost River Basin and could also be used to develop a potential future groundwater model.
Introduction
As its name implies, the Big Lost River has long been recognized for its extensive exchanges between surface water and groundwater. Debler and others (1931) described several “sinks” where the Big Lost River loses large quantities of streamflow to groundwater, and other areas of large gains. Crosthwaite and others (1970a, p. 2) stated that “a distinctive feature of the Big Lost River basin is the large interchange of water from surface streams into the ground and from the ground into surface streams.” This pattern of water exchange affects, and is affected by, groundwater and surface-water resource use in the Big Lost River Basin.
The Big Lost River Basin comprises extensive mountainous uplands and a narrow, intermontane valley in south-central Idaho on the northeastern boundary of the Snake River Plain (fig. 1). The valley contains several small communities centered around irrigated agriculture, ranching, and recreation. The Idaho National Laboratory (INL) lies southeast of the valley mouth. Streamflow losses from the Big Lost River recharge the eastern Snake River Plain aquifer through infiltration and basin underflow.
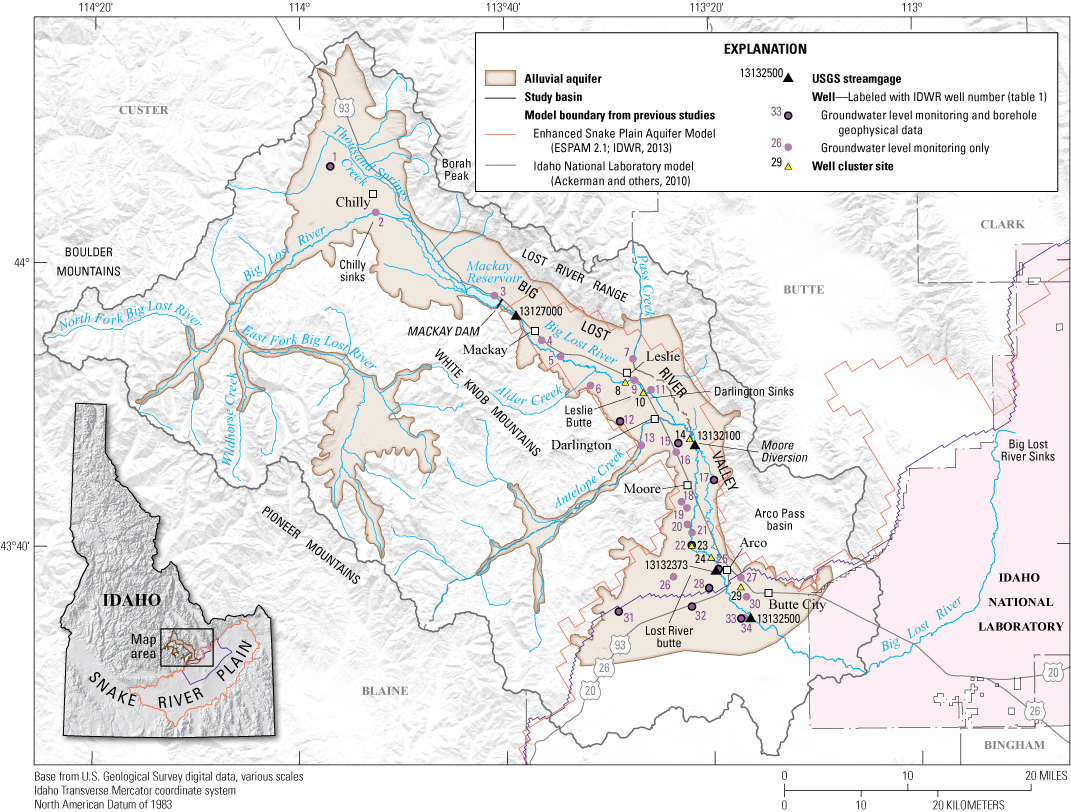
Location of study area in the Big Lost River Basin, south-central Idaho. IDWR, Idaho Department of Water Resources; USGS, U.S. Geological Survey.
In September 2016, the Director of the Idaho Department of Water Resources (IDWR) was petitioned to designate a Critical Ground Water Area in the Big Lost River Basin by water users expressing concerns about declining groundwater levels, declining streamflow in the Big Lost River, and drought (Bernal, 2016). The IDWR received a subsequent petition to designate a Ground Water Management Area (Broadie, 2017a; 2017b). Contemporaneous analysis by IDWR indicated statistically significant declining trends in groundwater levels in the basin (Sukow, 2017). Although both petitions ultimately were withdrawn, water users remain concerned about the condition and future of water resources in the Big Lost River Basin (Bernal and Broadie, 2017). The last comprehensive study of water resources in the basin was done in the late 1960s (Crosthwaite and others, 1970a; 1970b); thus, there is a need for an updated evaluation of water resources in the Big Lost River Basin to inform current water-management decisions.
Purpose and Scope
The purpose of this report is to describe an updated hydrogeologic framework for the Big Lost River Basin to help inform current water-management decisions. The hydrogeologic framework includes three major parts: (1) a conceptual description of the hydrogeologic units, (2) a three-dimensional hydrogeologic framework model of the hydrogeologic units and subunits, and (3) a description of groundwater occurrence and movement within the basin. This framework is based primarily on a compilation and interpretation of existing data and previous studies, including well-driller reports, geologic maps, and groundwater-level data collected by IDWR, as well as borehole geophysical data collected during this study. The three-dimensional hydrogeologic framework model and supporting hydrogeologic framework well database are available in a companion data release to this report (Zinsser, 2021). This hydrogeologic framework is the first of three planned reports on water resources of the Big Lost River Basin; subsequent reports are intended to present a water budget for the basin and to describe the results of a series of measurement events quantifying gains to and losses from surface water in the Big Lost River (Dudunake and Zinsser, 2021).
Study Area
The Big Lost River Basin encompasses mountainous uplands and an irrigated agricultural valley in south-central Idaho (fig. 1). The mountainous areas include the western face of the Lost River Range to the east, the White Knob Mountains to the west, part of the Pioneer Mountains to the far west, and part of the Boulder Mountains to the northwest. Most of the basin is mountainous rangeland; about 8 percent of the land area is agricultural land and 18 percent is forest. Altitude in the basin ranges from a maximum of 12,667 feet (ft) on Mount Borah, the highest point in Idaho, to a minimum of 5,240 ft near Arco, Idaho. Mean annual precipitation in the basin is 18.2 inches (U.S. Geological Survey, 2016).
The main geographic feature in the Big Lost River Basin is a long, narrow, northwest-trending, intermontane valley stretching from the headwaters of Thousand Springs Creek to south of Arco (fig. 1). The valley is fault-bounded on the eastern range front and contains extensive irrigation infrastructure, including the Mackay Dam and Reservoir and a system of canals. Landmarks and incorporated communities in the valley from north to south include Chilly, Mackay, Leslie, Darlington, Moore and Arco. The Big Lost River Valley specifically refers to the valley south of Mackay Reservoir. The drainage area contributing to U.S. Geological Survey (USGS) streamgage 13132500, located at the mouth of the Big Lost River Valley south of Arco, is 1,410 square miles (U.S. Geological Survey, 2016). Downstream from this streamgage, the Big Lost River arcs south and east through the INL until reaching the Big Lost River Sinks, where the surface water is lost to evaporation or percolates into the subsurface and recharges the eastern Snake River Plain aquifer. Streamflow through this full channel length is intermittent depending on precipitation and upstream irrigation demands. The eastern Snake River Plain aquifer is highly productive and consists of volcanic and sedimentary rocks. It is recharged through infiltration of precipitation, applied irrigation water, streamflow (including the Big Lost River), and groundwater inflow from tributary mountain basins (including the Big Lost River Basin) (Ackerman and others, 2006).
Irrigation is vital to agriculture and ranching in the Big Lost River Basin. The first water right was filed in 1879 by an early settler for a ranch north of Mackay. Copper mining boomed in the White Knob Mountains and small-scale, irrigated agriculture began in the valley between Mackay and Arco in the 1880s (Bottolfsen, 1926). Extensive irrigation infrastructure, including the construction of Mackay Dam and Reservoir, was developed in the Big Lost River Basin during the early 1900s under the Carey Act (Lovin, 1987). By 1931, Livingston (1931) attributed the “present economic distress” to the overdevelopment of irrigated lands during the relatively wet period from 1904 to 1918. To supplement inadequate surface-water supply, extensive groundwater-resource development occurred in the basin from 1950 to 1977 (Sukow, 2017). Surface-water and groundwater irrigation use continues as the predominant water use for counties in the study area (U.S. Geological Survey, 2021a). Thus, water-resource management for irrigation, streamflow, and other economic benefits remains a vital concern in the Big Lost River Basin (Bernal and Broadie, 2017).
Although the Big Lost River Basin is areally extensive, this hydrogeologic framework focuses on the alluvial aquifer in the Big Lost River Valley, with less attention paid to the alluvial aquifer in the valley north of Mackay Reservoir, in the upper Big Lost River valley (the primary drainage basin west of Chilly, hereinafter referred to with lowercase “valley” as that area is not a formally designated valley in the U.S. Board on Geographic Names), in the Antelope Creek valley, and the bedrock aquifer (fig. 1). This focus is largely driven by predominant water-use and water-management questions associated with the alluvial aquifer in the Big Lost River Valley. Additionally, few hydrologic data are available for bedrock, tributaries, and upland areas. This study follows the convention of Crosthwaite and others (1970a) by using “alluvial aquifer” to refer to the water-bearing, unconsolidated basin-fill sediments and using “major bedrock aquifer” to refer to water-bearing sedimentary rocks. The Big Lost River Valley as far upstream as the Mackay Dam is within the boundaries of the Enhanced Snake Plain Aquifer Model Version 2.1 (fig. 1; Idaho Department of Water Resources, 2013), and Quaternary basalts constitute the main aquifer in the eastern Snake River Plain with alluvium in tributary drainage basins (Whitehead, 1992). Thus, although groundwater in the Big Lost River Basin is part of and tributary to the eastern Snake River Plain aquifer, this study follows the nomenclature convention of Crosthwaite and others (1970a) to describe aquifer resources within the Big Lost River Basin.
Previous Work
Early work in the Big Lost River Basin focused on improving water delivery for irrigation and, therefore, necessarily considered patterns of surface-water losses and gains. Debler and others (1931) described major losses of water from the Big Lost River in sinks near Chilly, near Darlington, below the Moore Diversion, and below Arco. Losses also were reported in Antelope Creek upstream from its confluence with the Big Lost River. Water returns to the Big Lost River were described upstream from Mackay Reservoir, in the river upstream from Mackay, and upstream from the Moore Diversion (fig. 1). Notably, Debler and others (1931, p. 3) concluded that “the waters lost by Big Lost River in the Chilly sinks is returned to the stream above Mackay, and largely so above Mackay Reservoir” and that “waters lost in the Darlington Sinks merely pass through the Antelope Creek delta and return in part to the river in the vicinity of the Moore dam [diversion].” Debler and others (1931) suggested using by-pass channels or canals to avoid losses of surface water to groundwater in the informally named Chilly sinks below the Moore Diversion and across the Antelope Creek fan but advised against a canal to bypass water around the Darlington Sinks because of cost and uncertainties regarding patterns of loss and gain in this area. Early work by Stearns and others (1938) provided a relatively detailed description of surface-water and groundwater resources in the Big Lost River Basin as a part of a comprehensive volume describing the water resources of the Snake River Plain. This work included one map showing the depth to water table with depths less than 10 ft coinciding with areas of gaining surface water, and a second more detailed map of groundwater surface altitudes near the Moore Diversion (Stearns and others, 1938).
The most comprehensive characterization of water resources in the Big Lost River Basin was made by Crosthwaite and others (1970a). The study provided a hydrogeologic framework and water budget by drawing on a wide range of new and existing data, including precipitation data; streamflow records and measurements; geologic maps; groundwater levels in approximately 200 existing wells; geologic and hydrologic interpretation of six new wells; and seismic, gravity and resistivity surveys. Crosthwaite and others (1970a) defined five hydrogeologic units: (1) carbonate rocks, (2) noncarbonate rocks, (3) cemented alluvial deposits, (4) unconsolidated alluvial deposits, and (5) basalt. Carbonate rocks represented the major bedrock aquifer, and basalt locally yielded substantial quantities of water, but the principal aquifer was the unconsolidated alluvial fill in the valley. Geophysical data were used to define basin fill geometry; basin fill was interpreted to be 2,000–3,000 ft in the Thousand Springs Creek area, relatively thin (200–400 ft) near the Mackay Dam, over 5,000 ft east of Mackay, and progressively thinning to about 2,500 ft near Arco. Test wells described multiple water-bearing zones near Arco; groundwater generally was unconfined but there were some areas of perched water and some artesian wells. Variability in groundwater gradient, indicated by areas of gaining versus losing streamflows, was attributed to variations in the cross-sectional area of the valley, permeability of the alluvial fill, and the quantity of water in the aquifer. Like previous reports, Crosthwaite and others (1970a, p. 34) recognized the unusually extensive connection between surface water and groundwater, stating that “[s]urface and ground water are so closely related that neither can be considered as a separate source of supply.”
Water use in the Big Lost River Basin has been a vital concern for almost a century (Debler and others, 1931; Livingston, 1931). Although early reports focused on the efficiency and viability of surface-water infrastructure such as using canals to move water past the various sinks, by 1970 attention had turned to the potential effects of groundwater resource development. Crosthwaite and others (1970b) showed the value of conjunctive management of surface water and groundwater, suggesting that groundwater could be more heavily used in dry years but that use would need to be minimized in wet years to promote recharge because of the likelihood that increased groundwater withdrawals could interfere with streamflows and hence affect surface-water rights. Nonetheless, this era included substantial development of groundwater resources, with groundwater appropriations in the basin increasing from about 1 to 80 percent of the current total rights from 1950 to 1977 (Sukow, 2017). Correspondingly, reports in the 1980s and 1990s reflect an increasing need to understand the nature of the groundwater resource. Szczepanowski (1989) summarized previous work and advocated for conjunctive water-use management, Bassick and Jones (1992) completed an aquifer test and described groundwater flow and pumpage in the Big Lost River Valley, and Johnson and others (1991) quantified the detrimental impact to surface-water rights from increased groundwater use. A series of reports in the 1980s also documented the hydrologic and other effects of the 1983 Borah Peak earthquake (Stein and Bucknam, 1985; Wood and others, 1985). In a recent review of groundwater levels in the Big Lost River Basin, Sukow (2017) reported that many wells had statistically significant declining trends in groundwater levels since 1977. These trends were attributed to a combination of more frequently occurring dry years and increased use of groundwater for crop irrigation and were concurrent with decreased surface-water diversion use.
Approach
This study is based primarily on interpretation of existing hydrologic and geologic information, although some original borehole geophysical data were collected. In addition to a review of existing hydrologic and geologic literature, the development of the hydrogeologic framework was based on four components: (1) interpretation and digitization of well-driller reports; (2) three-dimensional modeling of hydrogeologic units and subunits using RockWorks17™; (3) analysis of groundwater levels, flow directions and gradients; and (4) collection and interpretation of borehole geophysical data.
Well Data
During the development of the hydrogeologic framework, a digital hydrogeologic framework well database of 621 wells in the Big Lost River Basin was created in RockWorks17™; the database includes well identifiers, location, total depth, borehole lithology, well construction, and other information. The primary source of this information was the IDWR database of scanned well-driller reports for wells in Idaho (Idaho Department of Water Resources, 2021a). Well drillers were not required to obtain a permit to drill a well or submit a report to IDWR prior to 1987; thus, the IDWR database mostly contains reports since 1987 and should not be considered comprehensive. About 1,800 reported wells from within the Big Lost River Basin initially were identified using the IDWR “Wells” geographic information system (GIS) dataset (Idaho Department of Water Resources, 2018). These wells were winnowed down to about 600 for inclusion in this analysis. Wells were selected foremost to achieve good spatial coverage across the basin; if multiple wells were available in an area, the well with maximum depth or most complete lithology and well construction descriptions was selected for inclusion. Additionally, groundwater-level monitoring wells in the Big Lost River Basin that were not represented in the IDWR well construction database were added to the hydrogeologic framework well database. When possible, construction and lithology information was retrieved from USGS well data archives and entered in RockWorks17™. However, 10 wells without complete lithology and well-construction information were included nonetheless in the hydrogeologic framework well database because these wells were used to monitor groundwater levels. The digital hydrogeologic framework well database is available in the companion data release (Zinsser, 2021).
Three-Dimensional Hydrogeologic-Framework Modeling
A three-dimensional hydrogeologic-framework model was developed to assist in visualizing and understanding hydrogeologic units in the basin. Well lithologies, interpreted from well-driller reports and entered in the digital hydrogeologic-framework well database, were assigned to hydrogeologic units and used to develop the three-dimensional model that represents the subsurface thickness and distribution of hydrogeologic units. Well locations were obtained from the IDWR “wells” GIS dataset (Idaho Department of Water Resources, 2018). Historically, well locations were reported to the 160-, 40-, or 10-acre tract level using the Public Land Survey System (PLSS); therefore, well locations may be approximate, although more recent wells tend to be more precisely located using Global Positioning System. Well land-surface altitudes were assigned using the USGS National Elevation Dataset 1/3 arc-second grid (approximately 33 ft or 10 meters [m]) digital elevation model; U. S. Geological Survey, 2020).
For modeling purposes, well lithologies were simplified to predominant type; for example, sandy clay was entered with clay as the primary lithology and sand as the secondary lithology. Where wells intercepted bedrock, the rock was interpreted based on the well-driller description, published geologic maps, and professional judgment. For example, “lava” was interpreted as basalt when found in the southern end of the Big Lost River Valley, and descriptions such as “sandstone,” “limestone,” and “shale” were simplified to the more generic term “sedimentary rock.” Each well lithology was then assigned to an overarching hydrogeologic unit.
There are 621 wells in the hydrogeologic-framework well database, of which 604 are real wells and 17 are synthetic wells. The 17 synthetic wells were generated to improve and constrain model representation of key bedrock features. Of these 17 synthetic wells, 13 are duplicates of real wells for which well-driller reports showed termination in several feet of bedrock; in the duplicate record, bedrock was extended to 25 ft total at the bottom of the well to improve modeling. For 4 of the 17 synthetic wells, 300-ft-deep sedimentary rock boreholes were generated in Leslie Butte to constrain model representation of this bedrock feature where no actual wells were located. In total, 608 wells (17 of which were simulated) were used to generate the three-dimensional hydrogeologic framework model (the 13 real wells that were duplicated with extended bedrock were not used to generate the model).
The three-dimensional hydrogeologic framework model was developed in RockWorks17™ using the highest probability algorithm with a horizontal search distance of 6,562 ft (2,000 m), a vertical search distance of 50 ft (15.2 m), and closest point declustering. Both feet and meters are reported because the model projection, Idaho Transverse Mercator, uses meters for horizontal location and well-driller reports use feet for depth. A polygon shapefile representing the alluvial aquifer surface trace was applied as a filter in RockWorks17™ prior to model processing such that the model was only developed for the area inside the polygon. A grid file representing the surface topography—with 656-ft (200-m) horizontal spacing and altitude derived from the National Elevation Dataset 1 arc-second grid (approximately 98 ft or 30 m) digital elevation model (U.S. Geological Survey, 2020)—was applied as a superface filter in RockWorks17™ to force the model to adhere to surface topography. Voxel size, where one voxel constitutes one model point as represented in three-dimensional space, matched the surface grid and was 656 ft by 656 ft by 25-ft deep (200 m by 200 m by 7.6-m deep).
Groundwater-Level Measurements
IDWR currently maintains a network of groundwater-level monitoring wells in the Big Lost River Basin, although the number and location of wells monitored have varied over time, and many of the wells previously were monitored by the USGS (table 1). Groundwater-level measurements therefore are available on the USGS website for older data (U.S. Geological Survey, 2021a), and on the IDWR website for older and newer data (Idaho Department of Water Resources, 2021b). These data have several uncertainties. Groundwater levels were not barometrically corrected prior to October 2019, but unvented transducer data have been corrected since. The land-surface elevations of the wells vary in provenance and thus the vertical datum cannot be verified for most of them. However, because groundwater-level altitudes vary by more than 1,000 ft from the upper to lower parts of the study area, the influence of uncertainties attributed to barometric effects and potential discrepancies between vertical datums likely are small relative to the overall gradient described. Groundwater levels within wells were not directly compared between corrected and uncorrected time periods.
Table 1.
Groundwater-level monitoring wells, Big Lost River Basin, south-central Idaho.[The “x” indicates that data are available or were collected. Name: As assigned by and used in this report. IDWR, Idaho Department of Water Resources. PLSS identifier: Identifier used by the Idaho Department of Water Resources and based on the township, range, and section of the well. USGS identifier: Unique numerical identifier that can be assigned by the U.S. Geological Survey to access well data (https://waterdata.usgs.gov/nwis). Abbreviation and symbol: PLSS: public land survey system; —, no data]
Groundwater-level data were used to simulate potentiometric surface altitude by kriging in ArcGIS™. Because wells varied in instrumentation and the frequency of measurements, manual measurement events were preferentially selected to provide maximum well coverage. For each year selected, the groundwater-level measurements recorded closest to April 1 or November 1, plus or minus 3 weeks, were used. Thus, data associated with an “April” surface for a given year may have been collected in March or April. Groundwater-level data also were used to evaluate vertical gradients in a series of shallow, well clusters, installed by IDWR in late 2019 as part of this project. Well cluster locations were selected by IDWR based on access and prior knowledge about areas where vertical gradients likely were important to understanding groundwater movement.
Geophysical and Borehole Video Log Data
Borehole geophysical data and borehole video files were collected from 10 groundwater-level monitoring wells using wireline logging tools. Geophysical logs were collected only in cased borehole sections because of the concerns of neutron and gamma-gamma logging tools being ensnared in uncased (open) wells. For each well, the measured depth to water, logging date, logging depth, and log type are identified in table 2. Wireline geophysical and borehole video equipment are owned and operated by the USGS INL Project Office. The USGS calibrates geophysical logging equipment and sensors annually or on an as-needed basis.
Table 2.
Summary of geophysical and video data collected from 10 wells in the Big Lost River Basin, south-central Idaho.[Geophysical data presented in this report were collected by the U.S. Geological Survey (USGS) based on well access. Borehole video files were run in both cased and open borehole sections. The “x” indicates that a measurement was collected and analyzed. Name: As assigned by and used in this report; site identifiers used by the USGS and Idaho Department of Water Resources (IDWR) are in table 1. DTW: Depth to water (DTW) is the measured depth to water taken from water-level measurement collected prior to logging the well. Date: Physical date on which the logs were collected. Logged depth: Logging depth reported from land surface to approximate bottom hole depth or where the tool started recording data. Log type: Type of geophysical measurement collected. Abbreviations and symbol: BLS, below land surface; mm-dd-yyyy, month-day-year; Temp., temperature; SpC., specific conductance; —, no data].
Borehole video files were used to confirm well depth, well-casing integrity, well-casing size, and well obstructions prior to collecting geophysical data. Natural gamma, neutron, gamma-gamma dual density, and fluid logs were collected and used to examine lithology and to approximate location and thickness of unconsolidated sediment and rock units. The natural gamma signal was used to identify changes in lithology by looking at signal changes. Neutron logs are a general indicator of hydrogen content and, when combined with natural gamma logs to determine lithology location, can be used to identify perched groundwater. The gamma-gamma dual density log was used to examine bulk density changes in the rock and unconsolidated sediment layers. Fluid specific conductance and temperature provide a general indicator for changing water chemistry when run in a vertical fluid column.
Well-driller reports, when available, were used initially to identify general borehole lithology for each well. However, this information was general or incomplete so geophysical logs were used to update lithology by estimating the location of unconsolidated sediment and rock contacts and changes in unconsolidated sediment layering where reasonable. Where well-driller reports were not available, well lithology was approximated based on well-driller reports for nearby wells (2 miles or less) and geophysical data. Wells without well-driller reports presented a higher degree of uncertainty when interpreting lithology. A more detailed description of the geophysical methods is presented in appendix 1.
Hydrogeologic Framework
The objective of this framework is to define the key hydrogeologic units in terms of geologic history, lithology, hydraulic properties, and groundwater occurrence and movement. Given the extensive interaction of surface water and groundwater in the basin, this framework also addresses hydrogeologic controls on such exchanges.
Geologic History
The geologic history of the Big Lost River Basin is complex, and much of it is tangential to the movement and occurrence of water in the basin. As such, the geologic history is presented briefly here (fig. 2) and the reader is referred to other authors for more extensive treatment. The Big Lost River Basin is a part of the Cordilleran orogenic belt and the Basin-and-Range province; it lies north of the Snake River Plain-Yellowstone hot spot track. The oldest rocks present are the Archean to Paleoproterozoic metamorphic rocks exposed in the Pioneer core complex at the western boundary of the basin (Link and Janecke, 1999; Lewis and others, 2012).
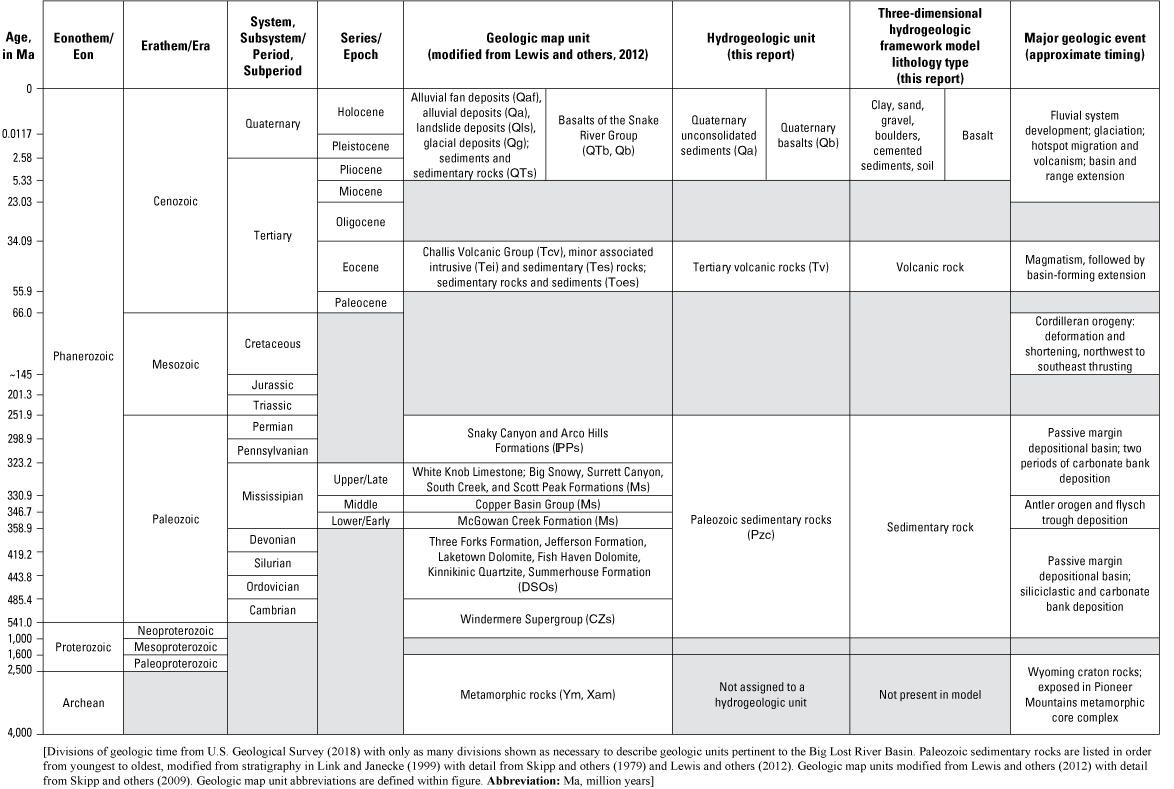
Correlation of geologic and hydrogeologic units of the Big Lost River Basin, south-central Idaho.
Most of the sedimentary rocks exposed in the White Knob, Pioneer, and Lost River Ranges were deposited in a series of successive, passive continental margin basins during the Paleozoic. During the late Neoproterozoic and Cambrian, siliciclastic rocks of the Windermere Supergroup were deposited southward into a passive basin; these rocks are exposed in the pebbly sandstones of the Wilbert Formation at the base of the Borah Peak horst, and on the western flank of the Pioneer core complex. An unconformable contact between these rocks and overlying Lower Ordovician sandstones of the Summerhouse Formation suggest an uplift event described as the Salmon River arch or Lemhi arch. Subsequent tectonic stability and depositional continuity began in the Middle Ordovician, represented geologically by the Kinnikinic Quartzite, and extended through the Devonian. Extensive carbonate banks developed during the Silurian, manifested in the dolomitic rocks of the Fish Haven and Laketown Formations. These rocks are exposed along much of the western face of the Lost River Range (Link and Janecke, 1999; Lewis and others, 2012). The Antler orogeny, characterized by presumed uplift to the west, occurred in the Late Devonian and Early Mississippian and is represented in the clastic foreland basin deposits of the McGowan Creek Formation argillite and conglomerates of the Copper Basin Group. In the Late Mississippian and Pennsylvanian, large carbonate bank complexes developed east of the Antler trough, eventually prograding to the west over the former trough. These carbonate bank deposits are extensive and include the White Knob Limestone and the Big Snowy, Surrett Canyon, South Creek, and Scott Peak Formations. These rocks are exposed most prominently in the upper Big Lost River, in the White Knob Mountains near Mackay (including abutting the Mackay Dam), near Leslie, along Antelope Creek, and at the southern end of the Lost River Range east of Arco (Link and Janecke, 1999; Lewis and others, 2012). Fine-grained siliciclastic and carbonate rocks deposited in the basin during the Pennsylvanian and Permian include the Snaky Canyon and Arco Hills Formations and are exposed most prominently at the mouth of Antelope Creek and in the Arco Pass Basin (Skipp and others, 1979; Link and Janecke, 1999; Lewis and others, 2012).
The development of the Cordilleran orogenic belt in the Mesozoic caused crustal deformation and shortening expressed in widespread folding and faulting. Regional thrust faults in the Pioneer Mountains in the western part of the Big Lost River Basin developed during this time, including the Hawley Creek-Copper Basin thrust plate that comprises the rocks exposed in the White Knob Mountains, the Lost River Range, and other ranges beyond the study area (Link and Janecke, 1999; Lewis and others, 2012). Extension and faulting occurred in the Tertiary prior to, continued synchronously with, and post-dated the Eocene Challis magmatic event. The Challis magmatic event was relatively short-lived but intense, producing lava flows, tuffs and intrusive rocks. The resulting Challis Volcanic Group includes some intrusive rocks that outcrop in the White Knob Mountains east of Mackay and minor sedimentary rocks throughout the Big Lost River Basin, but most of the rocks associated with the magmatic event are andesitic and dacitic lava flows and tuffs. These volcanic rocks unconformably overlie the Paleozoic sedimentary rocks and are prominent throughout the western side of the Big Lost River Basin in the Pioneer, Boulder, and White Knob Mountains (Moye and others, 1988; Link and Janecke, 1999; Lewis and others, 2012).
The eastern Snake River Plain represents the track of volcanism formed by the migration of the Yellowstone hot spot as the North American Continental Plate moved westward over a mantle plume during the late Tertiary and into the Quaternary (Pierce and Morgan, 1992). Basaltic shield volcanoes likely began as fissure eruptions and lasted as long as several months at a central vent or vent complex. These features define volcanic rift zones, which are linear arrays of volcanic landforms and structures. The volcanic rift zones developed roughly parallel to the basin-and-range structures at the boundaries of the eastern Snake River Plain (Kuntz and others, 1992). Craters of the Moon National Monument and Preserve is centered on the Great Rift, and the Arco-Big Southern Butte rift extends southeast from approximately Arco; flows from these rift zones mostly date to the Holocene (Kuntz and others, 1992; Skipp and others, 2009). Basaltic flows from the Craters of the Moon lava field are present in the far southwestern end of the study area, whereas the Lost River butte (the basalt knob west of Arco) and most of the basalts at the south end of the study area are associated with the Arco-Big Southern Butte rift (Kuntz and others, 1992; Skipp and others, 2009); these rocks are more broadly described as the Snake River Group. Uplift occurring with the Arco-Big Southern Butte rift created a new drainage divide and forced the west-flowing Pliocene Big Lost River east to its current course (Hodges and others, 2009).
Extension associated with Basin-and-Range faulting began in the late Tertiary, continues to the present, and generated the Lost River Range. Faults associated with this event generally strike northwest (Link and Janecke, 1999). The Lost River fault most recently ruptured in 1983 with the magnitude 7.3 (Richter scale) Borah Peak earthquake, originating at a depth of about 13 kilometers (km) and manifesting at the land surface with a fault scarp that visibly offsets alluvial deposits by more than 4 m. The Lost River fault is a normal fault for which the movement is down to the west (that is, the main valley is down-dropped relative to the Lost River Range) and has an estimated 2.7 km of structural relief (Stein and Bucknam, 1985). Thus, the present geometry of the main valley developed in the late Tertiary and is defined by Basin-and-Range extension and the Snake River Plain volcanism.
Less comprehensive geologic work has been done on the alluvial deposits of the Big Lost River Basin, but the timing of basin development suggests that alluvial fill in the main valley has been accumulating since the late Tertiary. Small cirque and valley glaciers were present in the Lost River Range and in the Pioneer and White Knob Mountains during the Pleistocene (Pierce and Scott, 1982). Pleistocene glacial deposits form most of the alluvial valley fill in the East and North Forks of the Big Lost River and in parts of the main valley northeast and southwest of Chilly (Lewis and others, 2012). The extensive river valley and alluvial fan gravels were deposited largely during the Pleistocene under markedly different climatic conditions and greater streamflow in both glaciated and non-glaciated drainages (Pierce and Scott, 1982). Although cementation occurs in some older alluvial deposits (Crosthwaite and others, 1970a), limited cementation has been described in more recently mapped alluvial fan surfaces and vertical sections (Patterson, 2006; Kenworthy and others, 2014). Alluvial deposits in the valley thus consist of glacial deposits in the upper Big Lost River and tributaries, extensive alluvial fans along the range fronts in the main valley, and fluvial stream deposits along the main axis of the valley. The cut and fill nature of streams and the subsequent reworking of glacial and alluvial deposits by stream migration has generated a highly spatially heterogenous distribution of sediments in the valley.
Adding to the complexity of the basin fill, basalt flows of the Snake River Group encroached from the south in the Quaternary, creating an area of interbedded basalt rocks and unconsolidated sediments in the southern end of the valley. Several researchers have suggested that basalt flows from the south have intermittently dammed the Big Lost River and produced a laterally continuous, fine-grained deposit in the subsurface near Arco (Stearns and others, 1938; Crosthwaite and others, 1970a). Detailed geologic mapping of the area shows at least three laterally extensive basalt flows that potentially could have blocked the Big Lost River for a period of time (Skipp and others, 2009).
Hydrogeologic Units
Given the complex geologic history of the Big Lost River Basin, the hydrogeologic units are defined broadly to simplify hydrogeologic interpretation and modeling, and to accord with the level of information available in the well-driller reports. The hydrogeologic units are shown in figures 2–3.
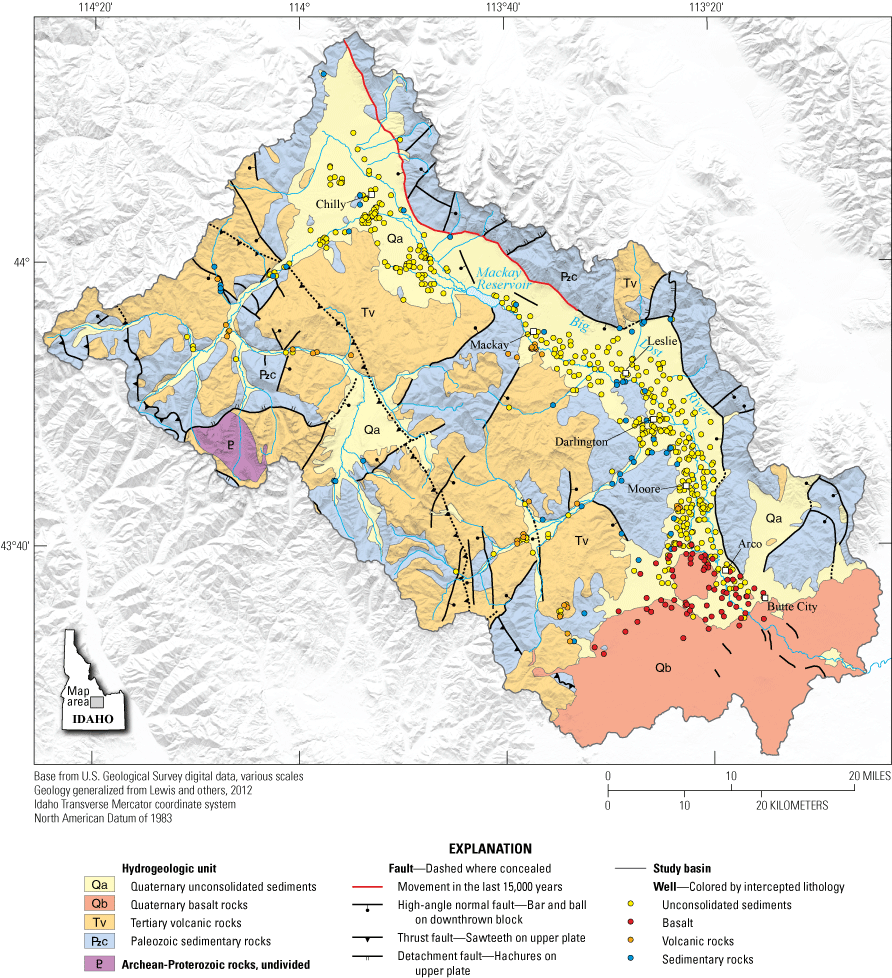
Hydrogeologic units, regional faults, and lithology intercepts for wells in the Big Lost River Basin, south-central Idaho. Geology is simplified from Lewis and others (2012).
Paleozoic Sedimentary Rocks
Paleozoic sedimentary rocks, with minor Neoproterozoic rocks, constitute the oldest hydrogeologic unit. The Paleozoic sedimentary rocks unit includes siliciclastic rocks such as the sandstones of the Windermere Supergroup and Summerhouse Formation, the Kinnikinic Quartzite, the argillite of the McGowan Creek Formation, and conglomerates of the Copper Basin Group. However, most of the rocks in this unit represent the extensive carbonate bank deposits from the Silurian (dolomitic rocks of the Fish Haven and Laketown Formations), Mississippian (White Knob Limestone and limestones of the Big Snowy, Surrett Canyon, South Creek, and Scott Peak Formations), and the Pennsylvanian to Permian (limestones of the Snaky Canyon and Arco Hills Formations) (figs. 2–3). Most of the porosity and permeability within the Paleozoic sedimentary rocks units likely are secondary in faults and fractures, but dissolution porosity and permeability may occur in some materials. Hydraulic conductivities derived from the literature for similar materials (limestone, dolomite, sandstone, and siltstone) range from 1.3×10-6 to 2,000 feet per day (ft/d) (Spitz and Moreno, 1996), where larger values are associated with fractured rocks (table 3).
Table 3.
Published ranges of hydraulic conductivity, transmissivity, and storage coefficient for hydrogeologic units in the Big Lost River Basin, south-central Idaho.[Data sources are noted in table footnotes. Abbreviations and symbol: ft/d, foot per day; ft2/d, feet squared per day; —, no data]
Well-driller reports and springs issuing from carbonate rocks indicate the importance of the Paleozoic sedimentary rocks unit as water-bearing at the margins of the alluvial aquifer and this unit likely is important to subsurface alluvial aquifer recharge at depth. Several prominent springs issue from Paleozoic sedimentary rocks in the Lost River Range, including Lower Cedar Creek and a series of springs along the Lost River fault northeast of Thousand Springs Creek. Crosthwaite and others (1970a) suggested that Hamilton Springs and Warm Springs, located near the head of Warm Springs Creek on the west side of the valley, discharge from carbonate units that outcrop nearby but are obscured by alluvium at the springs. Of the 604 real wells in the hydrogeologic framework well database, 48 intersect sedimentary rocks and of these, 39 are open to the sedimentary unit. Most of these 48 wells are located along the western margin of the main valley, in Antelope Creek valley, or in the upper Big Lost River valley, and a few are located on the eastern side of the valley (fig. 3).
Although the quality of lithology descriptions in the well-driller reports varies widely, the Paleozoic sedimentary rocks are not universally water-bearing. For example, well-driller reports describe (1) these rocks as having alternating water-bearing and fractured versus non-water-bearing and competent layers; (2) other rocks with long, non-water-bearing intervals; and (3) still other rocks with “caverns” producing no drilling fluid or cuttings returns. Hydraulic properties are heterogenous within the Paleozoic sedimentary rocks unit likely because of the diversity of geologic formations constituting the unit. Variability in hydraulic properties may be attributed to (1) differences in formation (for example, siliciclastic versus dolomite or limestone formations) that affect porosity; (2) fracture patterns at an unknown spatial scale (for example, alternating fractured and unfractured formations) that influence permeability; or (3) structural patterns (location of formations relative to faults) that dictate permeability. However, the well-driller reports and other available geologic information lack the detail to resolve these differences and as such, these rocks are grouped as one hydrogeologic unit. Generally, the Paleozoic sedimentary rocks unit is productive at the margins of the alluvial aquifer and likely is important to subsurface recharge in the alluvial aquifer.
Tertiary Volcanic Rocks
The Tertiary Challis Volcanic Group rocks, including the minor associated intrusive and sedimentary rocks, are grouped into the Tertiary volcanic rocks hydrogeologic unit (figs. 2–3). Composed of andesite, dacites and minor tuffs, this unit is assumed to have relatively low primary porosity and permeability, but fractures and faults likely provide secondary porosity and permeability and may be locally important for water production. Published hydraulic conductivities for similar rocks (fine-grained igneous rocks and tuff) range from 4.0×10-5 to 370 ft/d (Spitz and Moreno, 1996) (table 3).
Well-driller reports suggest that the Tertiary volcanic rocks unit may be important as a source of water to wells in small areas at the margins of the alluvial aquifer. Of the 604 real wells in the hydrogeologic framework well database, 43 intersect the Tertiary volcanic rocks unit and of these, 34 are screened or open to the volcanic rock. Most of these 43 wells are in the upper Big Lost River valley or its tributaries, along Antelope Creek, or south of Mackay. Based on highly variable lithologies described in the associated well-driller reports and regional geologic mapping (Skipp and others, 2009), the wells south of Mackay are in a fault zone (fig. 3). Therefore, the most important observation from this cluster of wells is the likely importance of the fault zone to the movement of water, rather than the lithology. In the Antelope Creek and East Fork Big Lost River valleys, multiple wells intersect the Tertiary volcanic rock unit, but most are screened in the Quaternary unconsolidated sediments above the volcanic rocks or in the uppermost, fractured bedrock. Thus, well-driller reports suggest that the Tertiary volcanic rocks hydrogeologic unit may be a locally important source of water, particularly associated with fault and fracture zones.
Quaternary Basalt Rocks
The Quaternary basalt rocks are an important hydrogeologic unit in the southern end of the Big Lost River Valley (figs. 2–3). Surface outcrops of basalt occur as far north as the Lost River butte west of Arco, but the northern subsurface extent of basalt is unclear because few deep (greater than 250 ft) wells have been drilled in the valley north of Arco. Subsurface intercepts of basalt occur about 5,000 ft north of the Lost River butte at an altitude of 5,034 ft (314 ft below land surface) in well ID 384237, and 3,300 ft north of the Lost River butte at an altitude of 4,960 ft (430 ft below land surface) in well IDWR-22. Using results from a surficial resistivity geophysical survey, Crosthwaite and others (1970a) suggested that volcanic rocks, either of the Challis Volcanic Group or the Snake River Group, may occur at depth north of Moore. However, no well-driller reports reviewed by this study confirmed the presence of basalt in the subsurface farther than 5,000 ft north of the Lost River butte.
The Quaternary basalt rocks hydrogeologic unit includes all basalt rocks in the study area not because they have the same hydraulic properties but because the well-driller reports lack the detail necessary to resolve hydraulic differences between the various basalt flows. Of the 604 real wells in the hydrogeologic framework well database, 74 intercept at least one basalt flow; however, wells open to the Quaternary basalt rocks often are open to multiple water-bearing zones of either alluvium or basalt. Basalt flows and alluvial layers above, below, or between basalt flows can be all water-bearing, which is broadly consistent with extensive hydrogeologic characterization of the basalt flows in the INL.
Ackerman and others (2006) define three basalt hydrogeologic units (numbered 1 to 3) underlying the INL; the upper two units are composed of Snake River Group rocks and, therefore, are pertinent to basalts of the Big Lost River Basin. Ackerman and others (2006) hydrogeologic unit 1 comprises relatively young, thin, densely fractured basalts with higher hydraulic conductivity and porosity than that of hydrogeologic unit 2 and with water transmitted through interflow zones. Ackerman and others (2006) hydrogeologic unit 2 is composed of relatively young, massive, less densely fractured basalts with lower hydraulic conductivity and porosity than hydrogeologic unit 1. The researchers suggest that sediment deposits between basalt layers likely affect hydraulic properties. For example, fine-grained sediment that fills cracks and voids may reduce hydraulic conductivity, but insufficient data were available to define hydraulic conductivity for the sediment layers (Ackerman and others, 2006). High-permeability interflow zones, which are highly fractured areas at the margins of basalt flows, are critical to hydraulic conductivity in the eastern Snake River Plain aquifer (Whitehead, 1992; Welhan and others, 2002). Although the basalt units of the Big Lost River Basin lack the detailed information needed to correlate them to the INL hydrogeologic units, detailed interpretation of the latter suggest that heterogenous conditions are likely to exist within and between the various basalt and alluvial layers in the Big Lost River Basin. Based on the properties of basalt hydrogeologic units described by Ackerman and others (2006), hydraulic conductivities in the Quaternary basalt rocks hydrogeologic unit can vary from 0.01 to 24,000 ft/d, with the highest values associated with interflow zones (table 3).
Quaternary Unconsolidated Sediments
Quaternary unconsolidated sediments comprise the most heavily used hydrogeologic unit in the Big Lost River Basin (figs. 2–3). Most (441) of the 604 real wells reviewed for the hydrogeologic framework well database are completed in unconsolidated sediments and do not intercept consolidated rock; these wells are in addition to the subset of wells that intercept consolidated rock but are nonetheless completed in sediments. As described in section, “Geologic History,” unconsolidated sediments in the basin have three general origins: glacial, alluvial, or fluvial. Although the locations of these sediments generally are predictable based on geologic mapping—glacial sediments are exposed in the upper reaches of the Big Lost River and tributaries, alluvial fan sediments occur at the valley margins, and fluvial stream sediments are deposited along the valley axis (Lewis and others, 2012)—the geologic origin of sediments largely cannot be resolved within the well-driller reports. Thus, unconsolidated sediments are assigned to the same overarching Quaternary unconsolidated sediments hydrogeologic unit and are subcategorized as a subunit according to dominant grain size (boulders, gravel, sand, clay) or other defining characteristics (cemented sediment, soil). Soils are unsaturated but serve an important function as infiltration passes through them to become recharge, and soils are frequently noted in well-driller reports; thus, soil was included as a subunit to fully represent ground conditions. Silt rarely was described in the reports and thus was grouped with clay because of similar hydrogeologic properties. Wells represented in the hydrogeologic framework well database are completed in every unconsolidated sediment subunit except soil. The Quaternary unconsolidated sediments unit comprises the alluvial aquifer.
The large range of grain sizes present in the Quaternary unconsolidated sediments hydrogeologic unit has a correspondingly large range of hydraulic conductivities. Published hydraulic conductivities for unconsolidated sediments encompassing variable sorting and grain size distributions are shown in table 3; hydraulic conductivity values range from 2.8×10-6 to 0.0028 ft/d for clay and from 28 to 2,800 ft/d for gravel (Fetter, 2001). Transmissivity and storage coefficient were calculated for the alluvial aquifer southwest of Moore in an aquifer test completed by Bassick and Jones (1992) and are shown in table 3. The aquifer was described as layered gravel, sand and clay with some degree of local confinement. Transmissivity ranged from 61,000 to 330,000 ft2/d, and storage coefficient ranged from 0.0006 to 0.02 (Bassick and Jones, 1992).
As described in section, “Geologic History,” the history of sediment deposition and volcanism suggests that subunits within the Quaternary unconsolidated sediment hydrogeologic unit are likely to be highly discontinuous vertically and laterally. However, some general patterns of permeability have been reported by previous investigators. Quaternary unconsolidated sediment subunits tend to be coarser (sand and gravel) and more well-sorted proximal to the Big Lost River, and more poorly sorted with more clay towards the valley margins. This pattern manifests in less draw-down for wells in the more permeable subunits in the central axis of the valley (Crosthwaite and others, 1970a), and greater draw-down, less permeability, and more frequent confining conditions at the margins of the valley (Crosthwaite and others, 1970a; Bassick and Jones, 1992). Confining conditions also are reported in well-driller reports for wells near Arco and may be associated with a fine-grained, laterally continuous confining layer deposited after basalt flows dammed the Big Lost River (Stearns and others, 1938; Crosthwaite and others, 1970a; Skipp and others, 2009).
Geophysical Results
The results from the borehole geophysics were difficult to interpret in detail for many wells because multiple casing strings and large-diameter wells muted the response of the geophysical tools. Additionally, unknown construction methods and limited or entirely missing lithology descriptions further complicated interpretation. The primary goal for the borehole geophysics was to help determine and define, if applicable, the presence of a laterally continuous, fine-grained confining layer. Based on the results of the geophysics, as well as the three-dimensional hydrogeologic framework model, confining layers in the Big Lost River Basin aquifer generally are spatially discontinuous. Thus, the borehole geophysical results primarily are helpful in resolving local-scale lithology and are discussed in sections, “Three-Dimensional Hydrogeologic Framework Model” and “Groundwater Occurrence and Movement,” as pertinent. The full borehole geophysical results are presented in appendix 1 or can be accessed online through the GeoLog Locator web page (U.S. Geological Survey, 2021b).
Three-Dimensional Hydrogeologic Framework Model
As described in section, “Approach,” the three-dimensional hydrogeologic framework model was developed with lithology data from 608 wells (fig. 4). The hydrogeologic framework well database and three-dimensional hydrogeologic framework model are available in the companion data release (Zinsser, 2021). The hydrogeologic framework model is used primarily to describe the spatial distribution of hydrogeologic units in the Big Lost River Basin. An overview of the model boundaries, model zones, well locations, and geologic section lines is provided in figure 4. Model zones were delineated to simplify display and discussion of the model; they do not imply or represent hydrologic boundaries. Geologic sections parallel to the long-axis of a valley are referred to herein as long sections; sections that cut across a valley (perpendicular to the long-axis) are referred to herein as cross sections. The geologic sections illustrate slices of the three-dimensional hydrogeologic framework model with intercepted wells shown to illustrate how accurately the model represented well lithology. However, the model slice depicted in the section was developed from multiple proximal wells according to the modeling algorithm search distances (6,562 ft horizontal, 50 ft vertical); thus, hydrogeologic units and subunits represented in section may or may not occur in the intercepted wells. The model also contains interpolation gaps in some areas owing to the sparseness of underlying well data; these gaps are depicted as blank areas in the geologic sections.
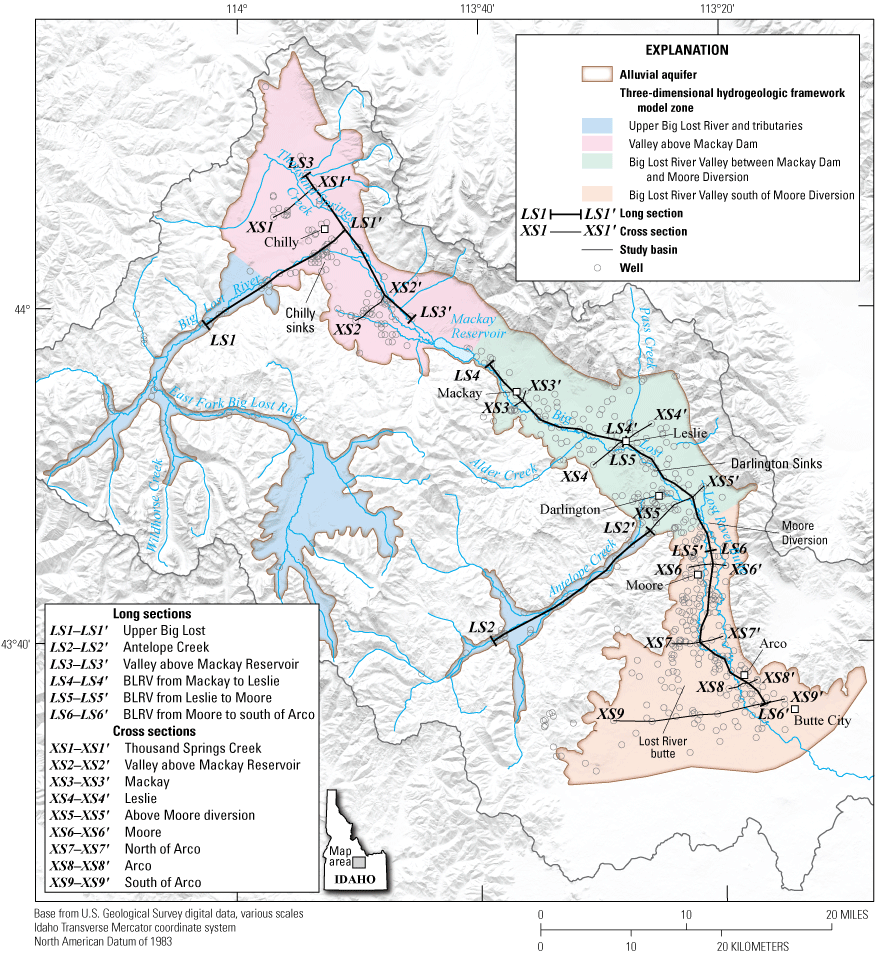
Overview of hydrogeologic framework model zone boundaries, wells, and geologic section lines, Big Lost River Basin, south-central Idaho. See figures 6–12 for long sections and cross sections identified in this figure.
Upper Big Lost River and Tributaries
The upper Big Lost River and tributaries primarily consist of upland areas dominated by the Paleozoic sedimentary rocks and Tertiary volcanic rocks units and narrow valleys with glacial and alluvial deposits constituting the thin Quaternary unconsolidated sediments unit. Correspondingly, the hydrogeologic framework model in the upper Big Lost River and Antelope Creek valleys has a greater volume of the Paleozoic sedimentary rocks and Tertiary volcanic rocks units (44 percent total), and more boulders (12 percent) in the Quaternary unconsolidated sediments unit, than in the main valley (table 4; fig. 5). Well data are sparse, but most wells are relatively shallow and are completed in the Quaternary unconsolidated sediments unit, although some deeper bedrock wells are completed in Paleozoic sedimentary rocks unit (fig. 6A–6B). In both the upper Big Lost River and Antelope Creek, the Quaternary unconsolidated sediments generally thicken and coarsen towards the mouth of the valley (fig. 6A–6B); this pattern is coincident with documented streamflow losses in the Chilly sinks and the Antelope Creek fan (Crosthwaite and others, 1970a; Clark, 2022).
Table 4.
Hydrogeologic unit volumes in each hydrogeologic framework model zone, Big Lost River Basin, south-central Idaho.[Hydrogeologic unit volumes by model zone are derived from the three-dimensional hydrogeologic framework model. Model zone boundaries are shown in figure 4. All numbers in table are percentages]
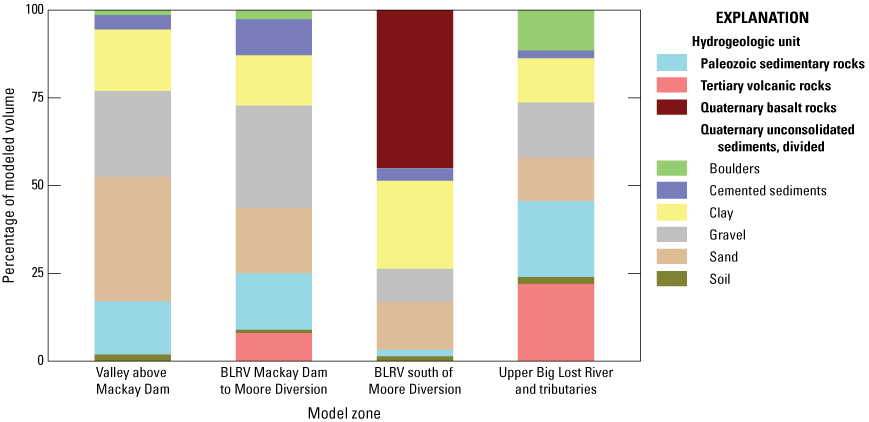
Hydrogeologic unit volumes in each hydrogeologic framework model zone, Big Lost River Basin, south-central Idaho. Boundaries of model zones are shown in figure 4. BLRV, Big Lost River Valley.
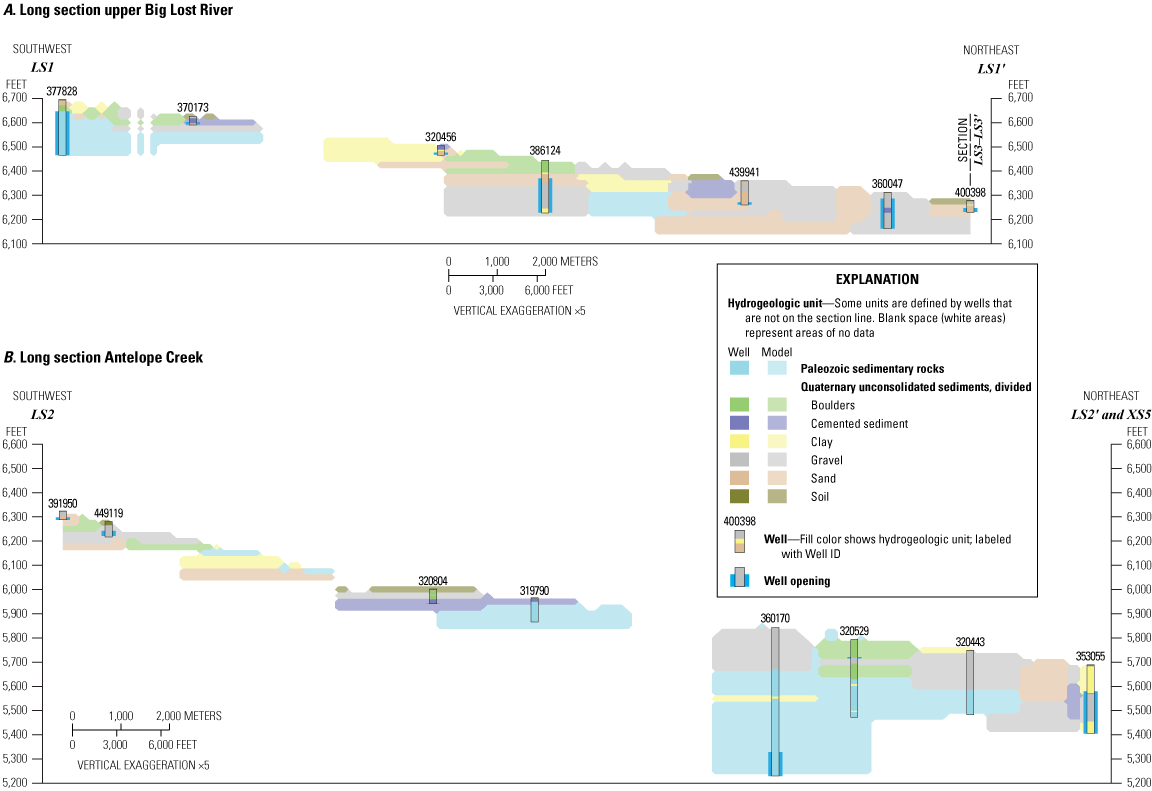
Geologic long sections of the hydrogeologic framework model in (A) upper Big Lost River and (B) Antelope Creek, Big Lost River Basin, south-central Idaho. Sections represent slices of the model with hydrogeologic units and subunits interpolated from wells within horizontal (6,562-foot) and vertical (5-foot) search distances. Locations of section lines are shown in figure 4.
Valley Above Mackay Dam
The valley above Mackay Dam represents an alluvial fill, structurally controlled extensional basin with the Paleozoic sedimentary rocks unit in the east and northwest range fronts and Tertiary volcanic rocks unit to the west. The Quaternary unconsolidated sediments unit is variable in depth and subunits are laterally discontinuous with variable grain size. This is shown in the model, with deeper Quaternary unconsolidated sediments with more clay north of Chilly; deeper and coarser sediments near the Chilly sinks; and shallower and coarser sediments approaching the Mackay Reservoir (figs. 4, 7). The area with fine subunits (clay) shown north of Chilly (figs. 4, 7A–7B) aligns with geophysical results (app. 1, well IDWR-1) and well-driller reports of confining conditions. Areas with coarse sediment subunits (sand and gravel) align well with documented streamflow losses in the Chilly sinks (figs. 4, 7A–7C) and streamflow gains upstream from the Mackay Reservoir (Crosthwaite and others, 1970a). Some deep wells in the Paleozoic sedimentary rocks unit occur on the eastern valley margin and near Chilly (figs. 4, 7A), indicating the importance of this unit to water production at the margins of the Quaternary unconsolidated sediments unit. Overall, the Quaternary unconsolidated sediments unit in this part of the model is predominantly coarse (59 percent gravel and sand subunits), although the clay subunit (18 percent) and Paleozoic sedimentary rocks unit (15 percent) also represent appreciable model volumes (table 4; fig. 5).
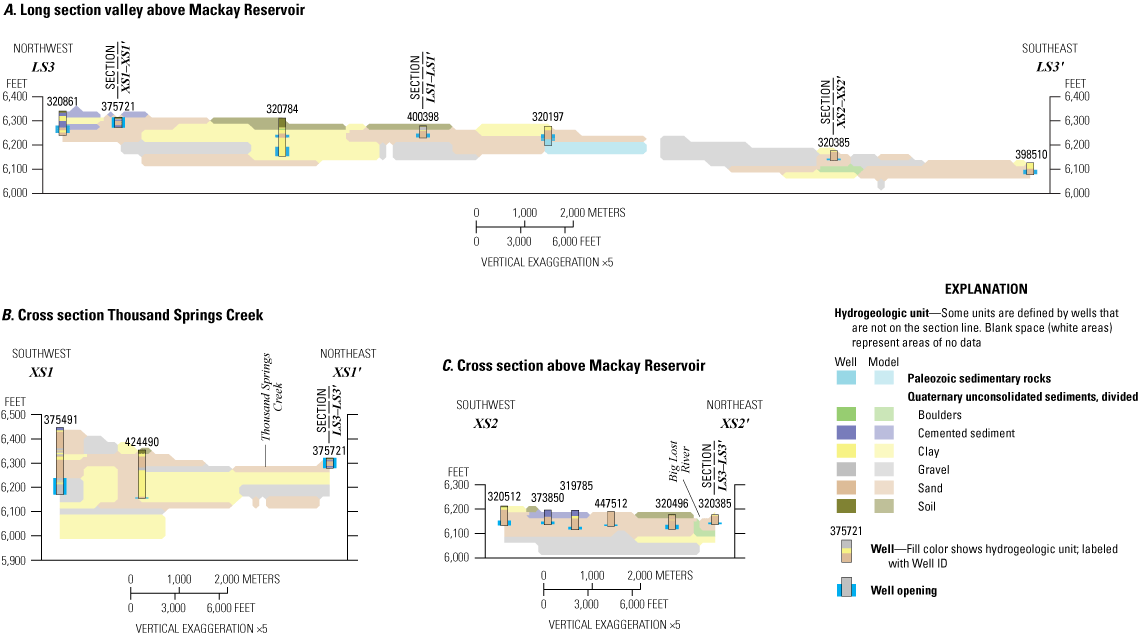
Geologic sections of the hydrogeologic framework model in (A) long section in the valley above Mackay Reservoir, (B) cross section in Thousand Springs Creek, and (C) cross section above Mackay Reservoir, Big Lost River Basin, south-central Idaho. Sections represent slices of the model with hydrogeologic units and subunits interpolated from wells within horizontal (6,562-foot) and vertical (50-foot) search distances. Locations of section lines are shown in figure 4.
Big Lost River Valley Between Mackay Dam and Moore Diversion
Below Mackay Dam, the Big Lost River Valley narrows substantially, with Paleozoic sedimentary rocks outcropping in the east and west, Tertiary volcanic rocks outcropping in the west, and thin Quaternary unconsolidated sediments in the valley bottom. The structurally controlled valley widens and deepens between Alder Creek and the Moore Diversion, with a thicker Quaternary unconsolidated sediment unit dominated by coarse subunits (gravel and sand). Prominent alluvial fans occur on the eastern range front throughout this part of the valley. The model represents the narrow, thin Quaternary unconsolidated sediments as shown in the sections through Mackay, with the Tertiary volcanic rocks unit on the west and Paleozoic sedimentary rocks unit on the east (fig. 8A–8B). The thin Quaternary unconsolidated sediments unit near Mackay has been associated historically with reported streamflow gains (Stearns and others, 1938; Crosthwaite and others, 1970a). The western Tertiary volcanic rocks unit and eastern Paleozoic sedimentary rocks unit shown in figure 8B. broadly agree with regional geology, but the modeled contact between the units is inconsistent with expected stratigraphy and was derived solely from the model extrapolation algorithm; it is unlikely to be an accurate representation of the subsurface geology.
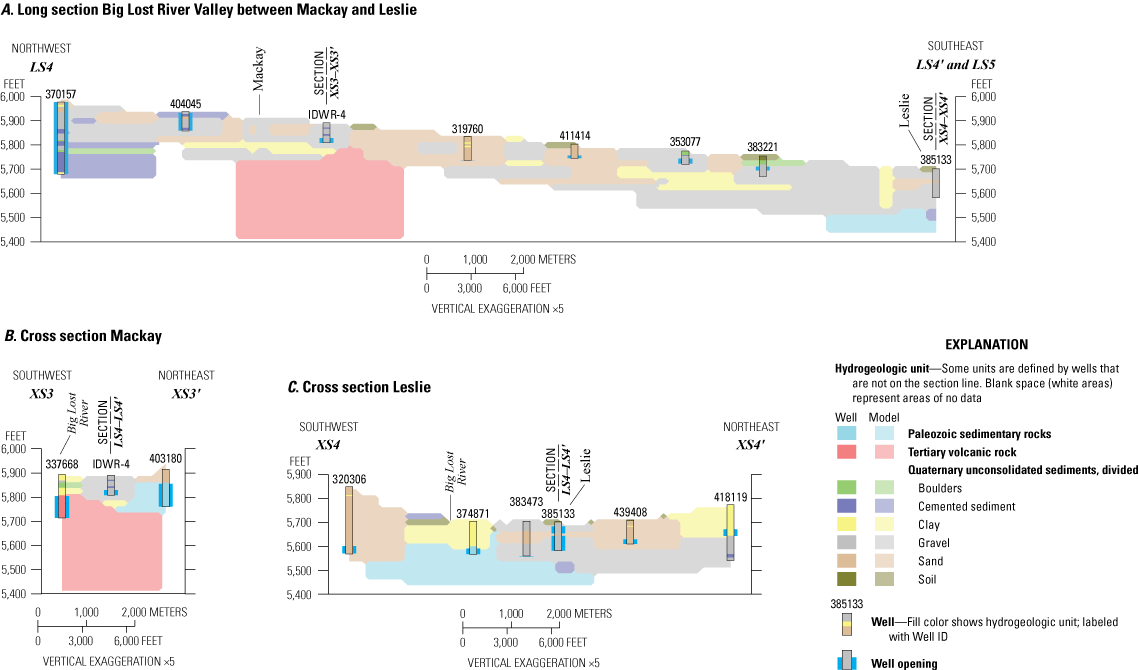
Geologic sections of the hydrogeologic framework model in (A) long section of the Big Lost River Valley between Mackay and Leslie, (B) cross section in Mackay, and (C) cross section in Leslie, Big Lost River Basin, south-central Idaho. Sections represent slices of the model with hydrogeologic units and subunits interpolated from wells within horizontal (6,562-foot) and vertical (50-foot) search distances. Locations of section lines are shown in figure 4.
Paleozoic sedimentary rocks also outcrop in Leslie Butte and are represented in the model at the head of the Darlington Sinks (figs. 4, 8A, 8C). The Darlington Sinks, known for large historical and contemporary streamflow losses (Crosthwaite and others, 1970a; Rice and Boyd, 2008; Dudunake and Zinsser, 2021), align with coarse subunits (gravel and sand) interpolated within the Quaternary unconsolidated sediments unit (figs. 8A, 8C, 9A). Fine subunits (clay) occur near the Moore Diversion (figs. 4, 9A–9B) where streamflow gains have been described historically (Debler and others, 1931; Crosthwaite and others, 1970a). Overall, this zone of the model has the highest proportion of the gravel subunit (29 percent) and lesser amounts of the sand (19 percent) and clay (14 percent) subunits within the Quaternary unconsolidated sediments unit, and the Paleozoic sedimentary rocks unit represents 16 percent (table 4; fig. 5). This zone also has the highest proportion of cemented sediments subunit (10 percent), which likely owes to the occurrence of wells in the large alluvial fans on the eastern range front.
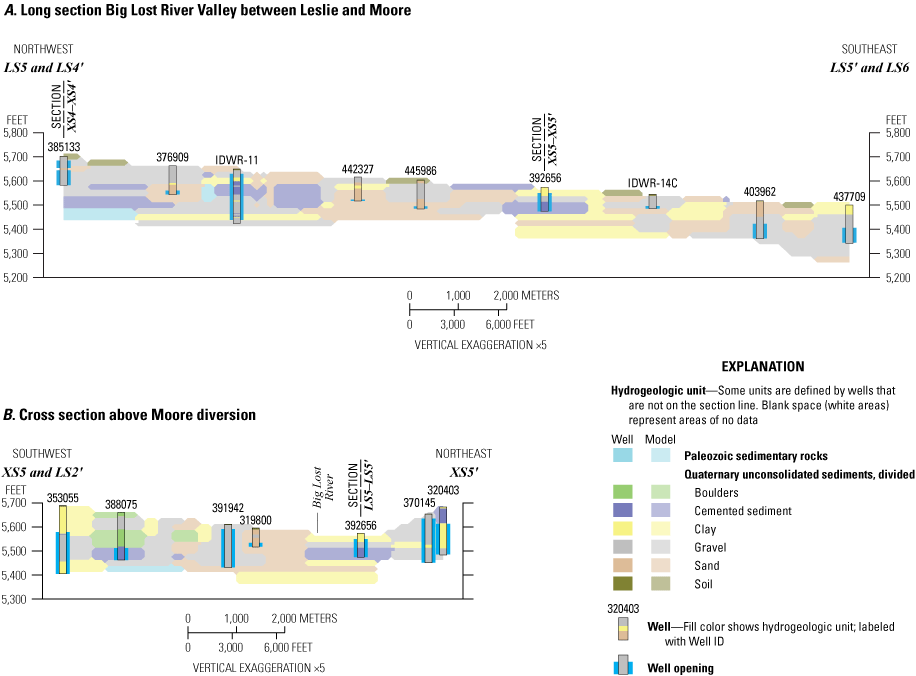
Geologic sections of the hydrogeologic framework model in (A) long section of the Big Lost River Valley between Leslie and Moore, and (B) cross section above the Moore Diversion, Big Lost River Basin, south-central Idaho. Sections represent slices of the model with hydrogeologic units and subunits interpolated from wells within horizontal (6,562-foot) and vertical (50-foot) search distances. Locations of section lines are shown in figure 4.
Big Lost River Valley South of Moore Diversion
The Big Lost River Valley south of the Moore Diversion is characterized by laterally discontinuous subunits of the Quaternary unconsolidated sediments unit and interbedding of this and the Quaternary basalt rocks unit as the valley opens onto the volcanic rift zones of the eastern Snake River Plain. Correspondingly, from Moore to just north of the Lost River butte, the model represents only the Quaternary unconsolidated sediments unit with predominantly coarser subunits (gravel and sand) in the long section and cross section (figs. 4, 10, 11A). Well-driller reports describe multiple water-bearing and non-water-bearing zones near Moore and long open intervals occur in wells in this area (fig. 10). The cross section through Moore (fig. 11A) also shows some clay on the western valley margin with wells open below the clay subunit; this is consistent with reports of a confining layer in this area (Bassick and Jones, 1992) and consistent with the conceptual understanding of coarse-grained sediments in the valley axis and fine-grained sediments at the margins (Crosthwaite and others, 1970a).
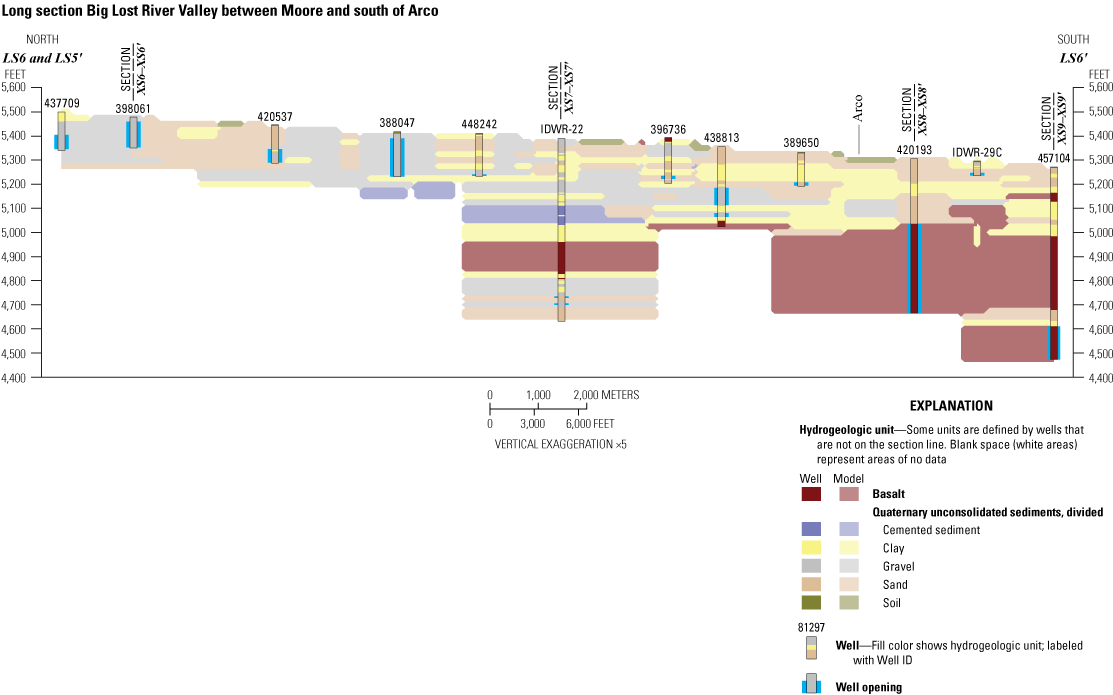
Geologic long section of the hydrogeologic framework model in the Big Lost River Valley between Moore and south of Arco, Big Lost River Basin, south-central Idaho. Section represents a slice of the model with hydrogeologic units and subunits interpolated from wells within horizontal (6,562-foot) and vertical (50-foot) search distances. Location of section line is shown in figure 4.
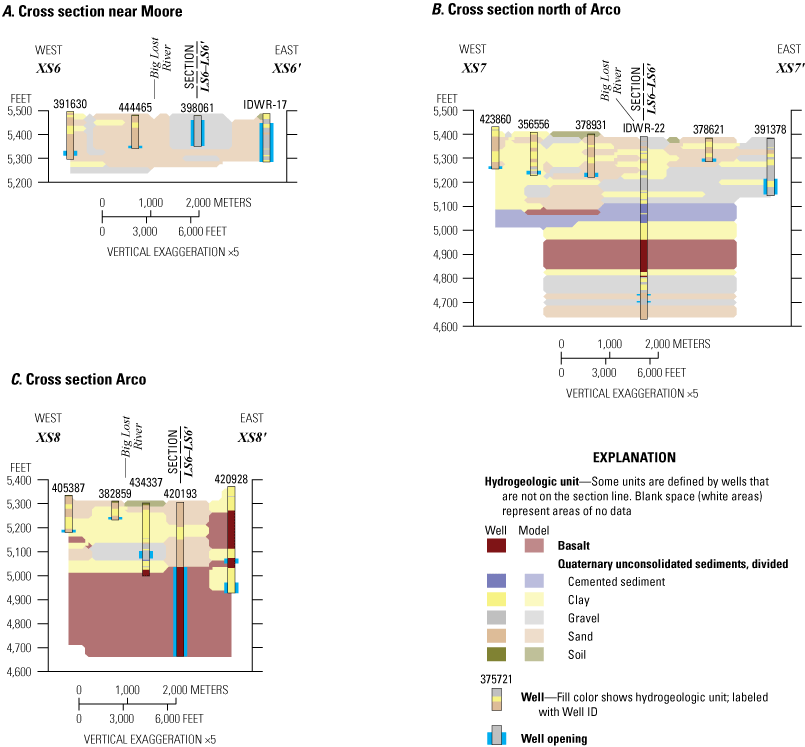
Geologic cross sections of the hydrogeologic framework model (A) near Moore, (B) north of Arco, and (C) near Arco, Big Lost River Basin, south-central Idaho. Sections represent slices of the model with hydrogeologic units and subunits interpolated from wells within horizontal (6,562-foot) and vertical (50-foot) search distances. Locations of section lines are shown in figure 4.
From approximately the Lost River butte to south of Arco, the hydrogeologic framework model represents interbedded Quaternary unconsolidated sediments and Quaternary basalt rocks units (figs. 4, 10). Basalt is first intercepted in the model at well IDWR-22 north of the Lost River butte; from that point south, basalt becomes increasingly predominant in the model. Wells are open in multiple units at various depths; this is visible in the long section (fig. 10) and cross section (figs. 11B–11C, 12) and is consistent with previous descriptions of water-bearing unconsolidated sediments and basalts in this area (Crosthwaite and others, 1970a). For example, wells are open in two distinct basalt flows and multiple separate sand and gravel subunits in the long section through Arco (fig. 10), and are open in at least three basalt flows and several sand and clay subunits in the cross section south of Arco (fig. 12). Clay subunits also become notably more dominant in the Arco area, which is congruent with previous reports of a fine-grained confining layer in this area (Stearns and others, 1938; Crosthwaite and others, 1970a). Overall, this model zone represents 45-percent Quaternary basalts rocks unit and 53 percent Quaternary unconsolidated sediments unit, with major subunits clay (25 percent), sand (14 percent) and gravel (9 percent gravel) (table 4; fig. 5).
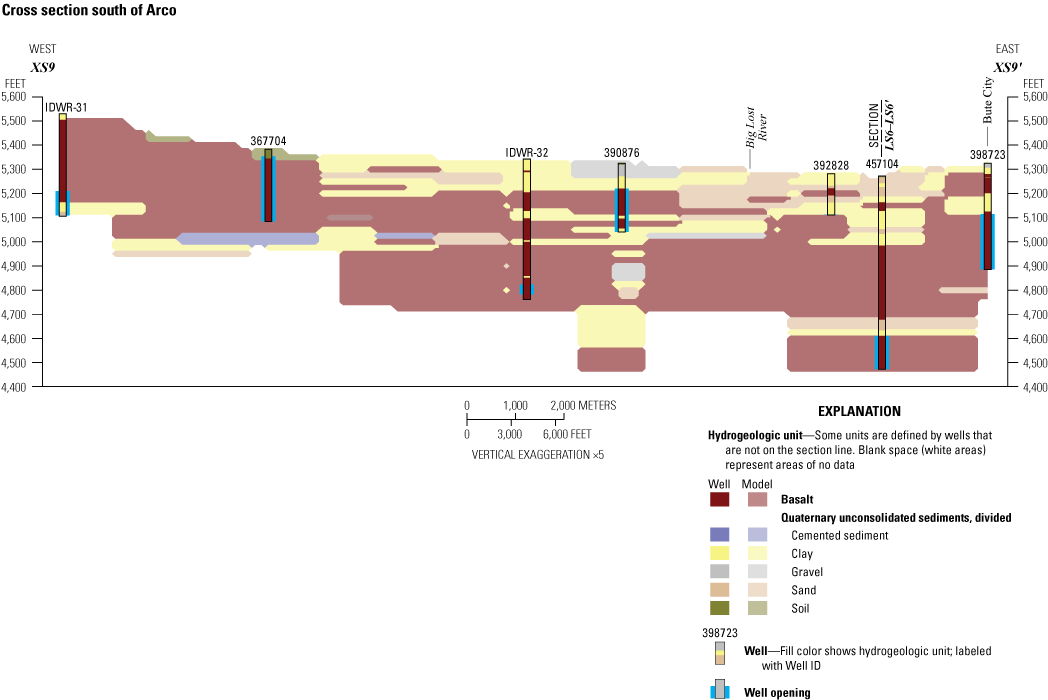
Geologic cross section of the hydrogeologic framework model south of Arco, Big Lost River Basin, south-central Idaho. Section represents a slice of the model with hydrogeologic units and subunits interpolated from wells within horizontal (6,562-foot) and vertical (50-foot) search distance. Location of section line is shown in figure 4.
Model Limitations and Uncertainties
The three-dimensional hydrogeologic framework model is limited by the quality of the underlying well data, generalization of the hydrogeologic units, and model parameters. Well-driller reports introduce multiple types of uncertainty into the model. As described in section, “Approach,” substantial uncertainty exists for the precise location of older wells. Although care was taken during digitization to remove wells that had obviously incorrect locations, more subtle errors may persist, and many wells are located only as precisely as the center of the nearest quarter section. Distribution of wells also varies; limited wells were available in tributaries to the Big Lost River and parts of the upper Big Lost River valley, and much greater well density was available in the valley south of Mackay but coverage at the margins of the valley was still limited.
The quality of lithology descriptions varied widely between well-driller reports and was necessarily interpreted and simplified for representation in the model. Contacts between hydrogeologic units and subunits should be considered approximate, and rotary drilling methods can cause underestimation of fine-grained sediments. The use of simplified hydrogeologic units causes transitions between units and subunits to be abrupt whereas they may be more gradual. As discussed in the “Hydrogeologic Units” section, substantial differences in hydraulic properties within hydrogeologic units almost certainly exist but cannot be resolved with the level of detail available in well-driller reports and other sources. Ideally, the three-dimensional hydrogeologic framework model would fully represent bedrock geology, faults, and aquifer geometry. However, wells (and hence well-driller reports) are heavily concentrated in the upper 250 ft of the alluvial aquifer and represent small-scale alluvial units, whereas aquifer geometry is best defined by the geophysical results reported in Crosthwaite and others (1970a) but represents thousands of feet, with no information to inform aquifer properties in between. Likewise, bedrock geologic structure, stratigraphy, and contacts can be broadly inferred from statewide and regional geologic mapping (Skipp and others, 2009; Lewis and others, 2012), but wells intercepting bedrock are limited and, thus, representation of bedrock in the hydrogeologic framework model is poor.
The three-dimensional hydrogeologic framework model is subject to the limitations of the underlying modeling algorithm and the decisions of the modeler. In the extensively faulted Big Lost River Basin, the highest-probability algorithm produced more geologically plausible interpretations with a more robust statistical basis than the other algorithm options. This algorithm nonetheless is sensitive to the search distances (6,562 ft or 2,000 m horizontal; 50 ft or 15.2 m vertical); these distances were selected to be approximately twice the average borehole spacing (about 3,281 ft or 1,000 m) and twice the vertical step (25 ft or 7.6 m), and were based on trial and error. Limited horizontal and vertical extrapolation distances are reasonable in the highly discontinuous alluvial sediments but vary in performance for describing bedrock. The 25-ft (7.6-m) vertical step likely is too coarse to accurately represent some small-scale, important features such as local confining layers while also being too fine for practical representation in groundwater flow models. The horizontal extrapolation distance is conservative for connecting the basalt units around Arco (and fails to plausibly connect basalt at depth in well IDWR-22 with the basalt in wells in Arco), but a greater horizontal extrapolation distance tended to produce implausible bedrock projections for the Tertiary volcanic and Paleozoic sedimentary rock units in other parts of the basin. Thus, the selected vertical and horizontal search distances represent reasonable albeit imperfect compromises between modeling objectives.
Groundwater Occurrence and Movement
Groundwater Occurrence
Based on the lithologies and depths of the wells in the hydrogeologic framework well database, groundwater resources are most heavily used in the Quaternary unconsolidated sediments unit within 250 ft of the land surface; this unit comprises the alluvial aquifer. The Paleozoic sedimentary rocks and Tertiary volcanic rocks units are important to water production and recharge at the margins of the alluvial aquifer, and the Quaternary basalt rocks unit is important to water production at the southern end of the Big Lost River Valley, but less data are available for these units than for the Quaternary unconsolidated sediments unit. Thus, most of the discussion of groundwater occurrence and movement focuses on the alluvial aquifer of the Quaternary unconsolidated sediment unit.
Although groundwater-level monitoring data exist for the Big Lost River Basin and can be used to describe regional groundwater movement in some parts of the valley (see section, “Groundwater Altitude and Flow Direction”), the coverage of these data is relatively sparse for the scale of the basin (fig. 1). Therefore, the depth to the top of the screened, perforated, or open interval (described herein as open or opening) for wells in the hydrogeologic framework well database was used to describe the general occurrence of water-bearing zones across the study area (fig. 13). Although the well opening data provided the best spatial coverage of water-bearing zones, these data have limitations and should not be understood as the top of the aquifer, nor as a potentiometric surface. For example, well-driller reports describe water found tens of feet before screen or perforations were installed in some wells, other wells are open solely at the bottom, and still other wells are open over long intervals (greater than 100 ft). The depth to screen therefore is deeper than the top of the aquifer in some places, may be shallower than a seasonally fluctuating water table in other places, and is a poor proxy for the potentiometric surface where wells are screened below confining layers. Additionally, domestic wells frequently are shallower with shorter screen intervals than irrigation wells, and wells often are drilled deeper over time, but well types and development date were reasonably evenly distributed across the basin and thus not expected to overly influence the results. Therefore, although it is an imperfect proxy, depth to well opening for the 604 real wells in the hydrogeologic framework well database was used to provide some insight into the general depth to water-bearing zones at a spatial resolution not possible using the groundwater-level monitoring wells.
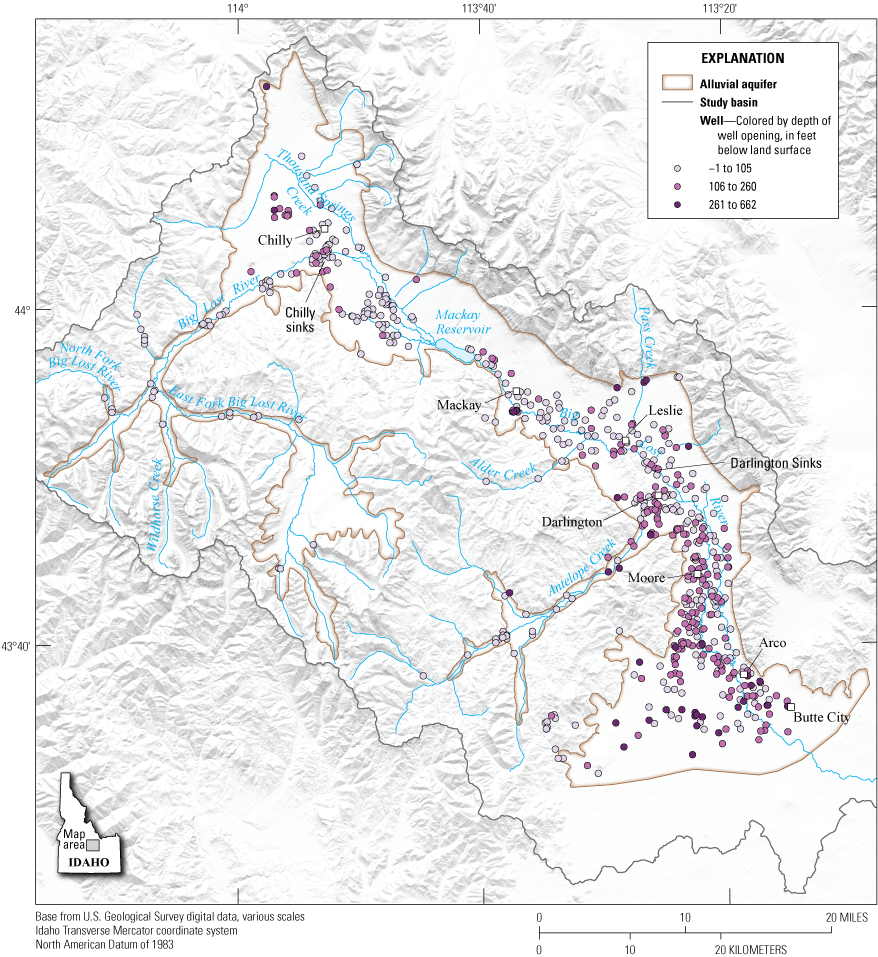
Depth to top of well opening for wells in the hydrogeologic framework well database, Big Lost River Basin, south-central Idaho.
Although wells are limited in the tributaries to the Big Lost River (East Fork and North Fork Big Lost River, Wildhorse Creek, Antelope Creek) and the valley above Chilly, the existing data suggest that groundwater is relatively shallow in these areas, with well intervals open less than 105 ft below land surface (fig. 13). Other areas with relatively shallow open intervals agree with historical accounts of areas with gaining streamflows, including above the Mackay Reservoir, between the towns of Mackay and Leslie, above the Moore Diversion, and near Arco. Similarly, areas of historical sinks align with moderate depth well openings (105–260 ft) in the Darlington Sinks but not in the Chilly sinks, where well openings were shallower (less than 105 ft). Some areas with deep well openings (261–662 ft) include wells northwest of Chilly, and wells open in bedrock at the valley margins south of Mackay, near Pass Creek, in the narrow part of Antelope Creek valley coincident with several mapped faults (Skipp and others, 2009), and south of Arco (fig. 13).
Groundwater Altitude and Flow Direction
Groundwater movement through an aquifer occurs as water flows from areas with higher hydraulic head to areas with lower hydraulic head; these head distributions are described by potentiometric surface maps. The IDWR maintains a network of groundwater-level monitoring wells in the Big Lost River Basin (table 1); these groundwater-level data were used to develop potentiometric surface maps to describe general groundwater movement between approximately Mackay and Arco. Because of the existence of confining layers in some parts of the valley, potentiometric surfaces are used here to be inclusive of both confining and non-confining conditions. The groundwater-level data, and hence the potentiometric surfaces, have some important limitations. For example, only about 24 wells have been semi-regularly measured over time, only three monitoring wells are located north of Mackay, and well completion depths and construction methods vary. Furthermore, wells may be open to multiple permeable zones, or screened in a locally confined layer. Several wells in the long-term monitoring network were removed from this analysis because they are screened in deep basalt (well IDWR-32) or in deep Quaternary unconsolidated sediments below basalt (wells IDWR-22 and IDWR-27) or indicate reservoir surface-water levels (well IDWR-3). Because the relation between groundwater and surface water is not known for all river sections, and because where known, relations can vary seasonally and over time (Dudunake and Zinsser, 2021), contours were generated without respect to surface-water altitudes.
Groundwater flow generally mimics topography, with flow overall down-valley to the south (fig. 14). Consistent with previous reports (Crosthwaite and others, 1970a; Bassick and Jones, 1992), the groundwater gradient flattens, around 15 feet per mile (ft/mi), between Darlington and Moore as the valley widens. The groundwater gradient steepens, around 65 ft/mi, at the mouth of the Big Lost River Valley as the alluvial aquifer transitions to the deeper, basalt aquifer in the eastern Snake River Plain (Stearns and others, 1938; Whitehead, 1992; Ackerman and others, 2010). Despite inconsistencies in the monitoring well network over time, 50-ft contour intervals of the potentiometric surface altitude are similar between April 1968 and April 2019, although the overall potentiometric surface altitude has lowered (that is, lines of equal potential occurred farther upgradient in 2019 than in 1968) from approximately Leslie through Moore (fig. 14).
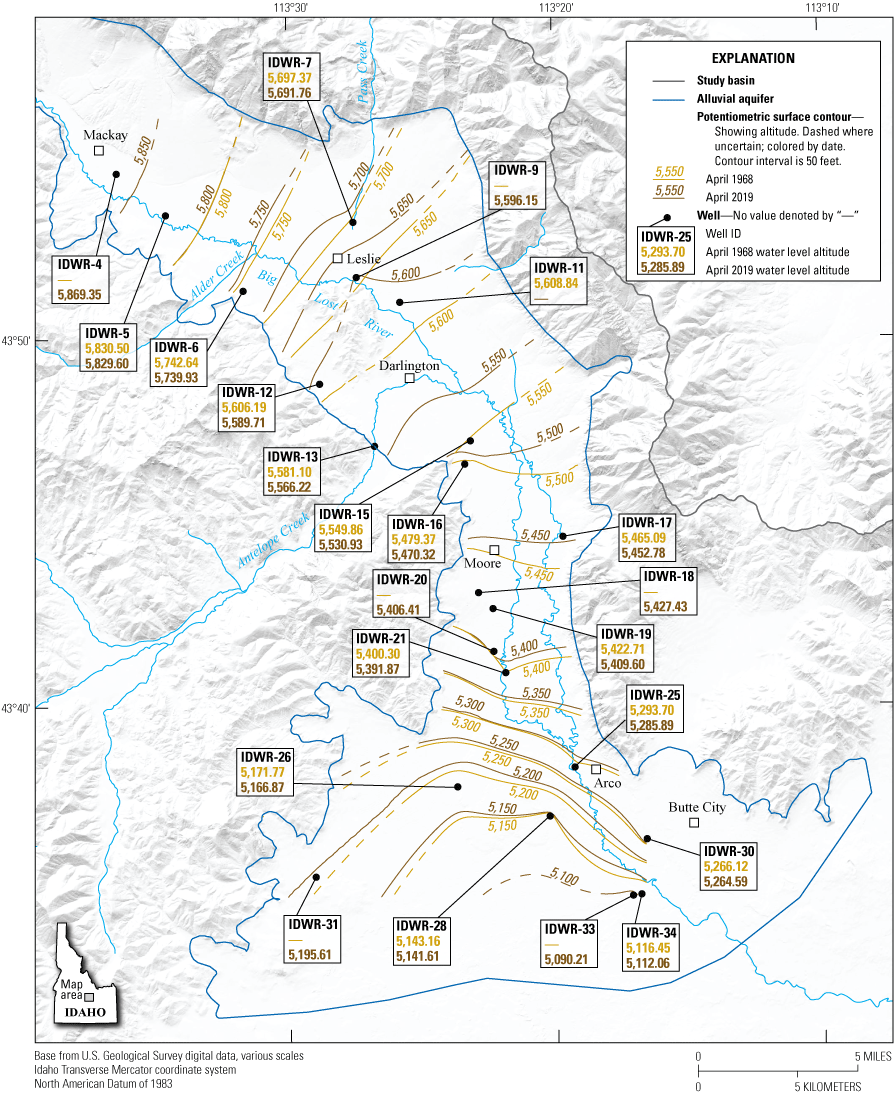
Groundwater potentiometric surface altitude, Big Lost River Valley, south-central Idaho, April 1968 and April 2019. Vertical datum could not be verified for all well altitudes.
Time-series data showing potentiometric surface altitude for select wells in the Big Lost River Valley show interannual fluctuations that likely respond to annual precipitation, recharge and irrigation demand, and decadal variations that likely are influenced by climatic cycles (fig. 15). Consistent with the potentiometric surface altitude map (fig. 14), the wells near Mackay (well IDWR-5) and south of Arco (well IDWR-33) show dampened fluctuations (11- and 23-ft maximum change, respectively), whereas wells between the Moore Diversion and Arco (IDWR-16 to IDWR-21) show larger fluctuations (71–109 ft maximum change; fig. 15). Wells south of Darlington show similar responses over time, suggesting that despite the presence of locally confining conditions in some areas, at least the upper 200 ft of the Quaternary unconsolidated sediments unit is hydraulically well connected overall.
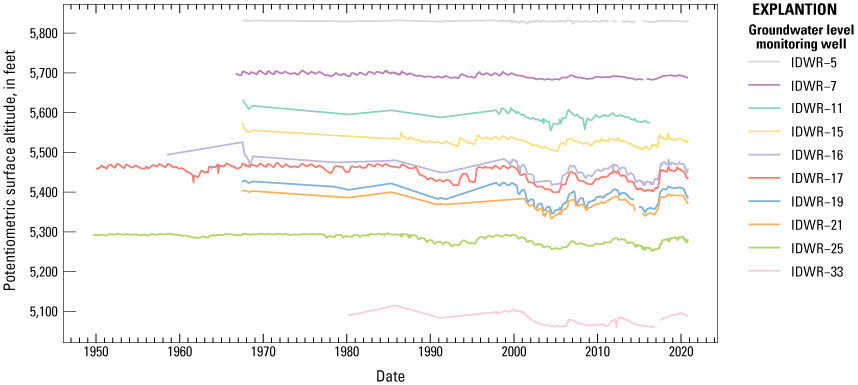
Groundwater potentiometric surface altitudes for select wells, Big Lost River Valley, south-central Idaho, 1949–2020. Vertical datum could not be verified for all well altitudes.
Fluctuations in potentiometric surface altitudes also are common seasonally, although the direction of change can vary. For example, 2017 was a wet year for the basin (Natural Resources Conservation Service, 2017; Clark, 2022), and potentiometric surface altitudes in the basin showed increases (that is, lines of equal potential moved downgradient) during April–November 2017 (fig. 16). An increase in the potentiometric surface is particularly apparent between Leslie and Arco, and suggests that natural recharge, incidental recharge from irrigation, and (or) intentional aquifer recharge activities occurring during irrigation season can increase storage in the aquifer over months. Alternately, 2020 was a dry year for the basin (Natural Resources Conservation Service, 2020), and potentiometric surface altitudes declined during April–November 2020 (fig. 17), particularly between Leslie and Arco. These fluctuations suggest that drawdown on the aquifer can be substantial over the course of an irrigation season and that hydrogeologic units in this area are highly transmissive and well-connected. Substantial changes in groundwater storage in this part of the basin are consistent with results from Clark (2022) that indicate incidental recharge is important to the groundwater budget in this area. Notably, potentiometric surface altitudes around Mackay and south of Arco changed relatively little seasonally or interannually; this may be a function of less groundwater demand in these areas and (or) aquifer characteristics such as volume, permeability, and zonation.
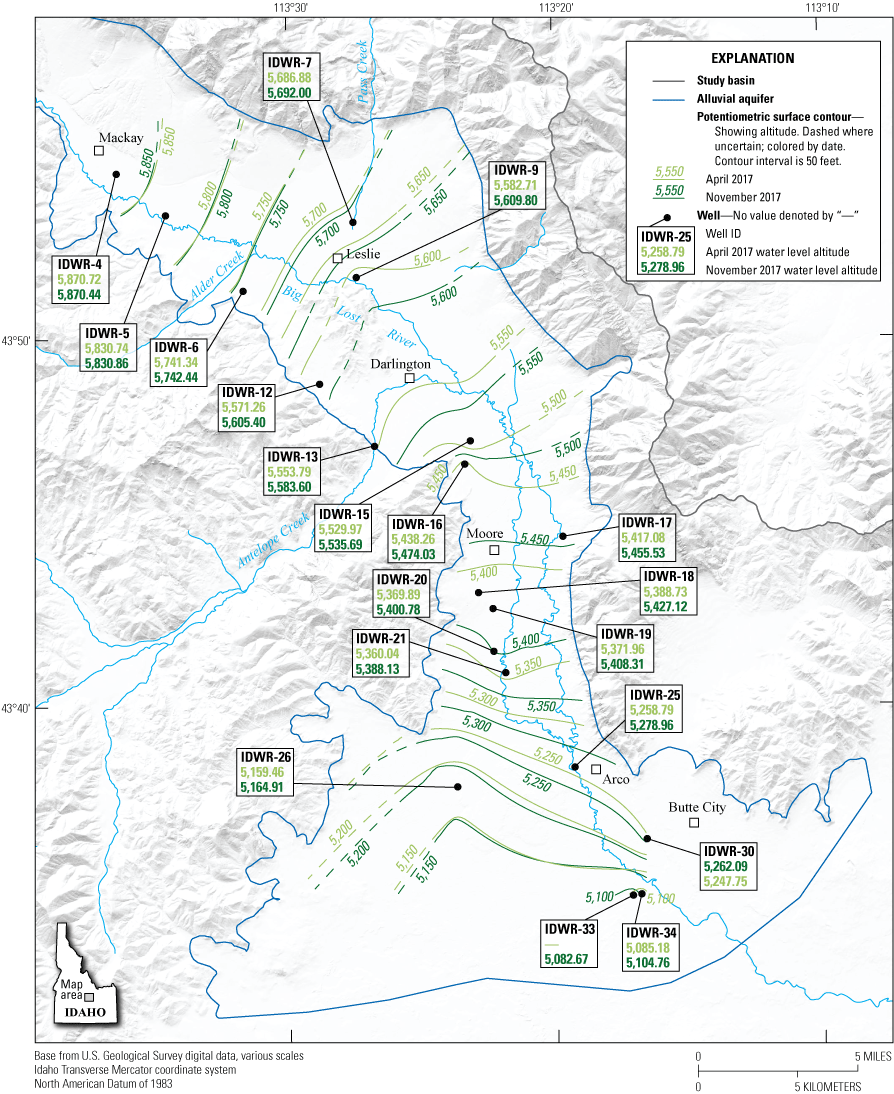
Groundwater potentiometric surface altitude, Big Lost River Valley, south-central Idaho, April and November 2017. Vertical datum could not be verified for all well altitudes.
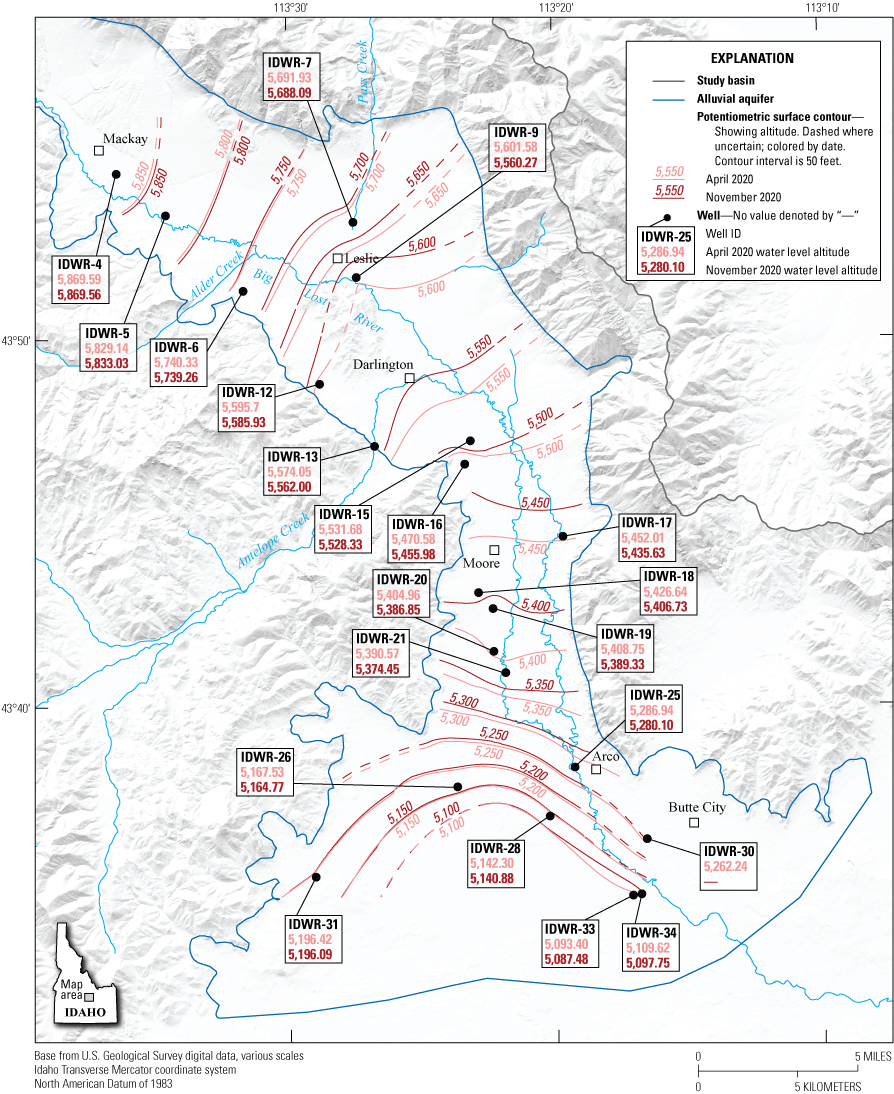
Groundwater potentiometric surface altitude, Big Lost River Valley, south-central Idaho, April and November 2020. Vertical datum could not be verified for all well altitudes.
Information about vertical hydraulic gradients in the Big Lost River Basin is limited. To address this data gap, a series of shallow (20-, 40-, and 60-ft) well clusters were installed by IDWR in late 2019 (fig. 1); the data therefore have a short period of record but nonetheless are helpful in describing vertical gradients in key locations. At well cluster site IDWR-8, located immediately north of Leslie Butte and near the Darlington Sinks, water consistently was present only in the mid-depth (40-ft) well, which suggests limited groundwater adjacent to Leslie Butte in 2019 and 2020 (fig. 18). Also occurring in the Darlington Sinks, the shallow (20-ft) well at well cluster site IDWR-10 due east of Leslie Butte was dry in late 2019 and 2020, but potentiometric surface altitudes in the 40- and 60-ft wells indicated a large downward gradient, although responses were somewhat decoupled between the two wells (fig. 18). At well cluster sites IDWR-14, IDWR-23, and IDWR-29, potentiometric surface altitudes at each depth are within inches of each other and responses are similar, suggesting no or little vertical gradient in these areas during late 2019 and 2020 and limited attenuation within 60 ft of the land surface (fig. 18). The 20- and 40-ft wells at well cluster site IDWR-24 have nearly identical potentiometric surface altitudes, whereas the 60-ft well potentiometric surface altitude is lower than the shallow wells; this indicates a downward vertical gradient and some attenuation at the lowest well (fig. 18). Notably, the southernmost well cluster sites (IDWR-23, IDWR-24 and IDWR-29) show rapid increases in potentiometric surface altitude in March 2020; this rapid recharge was synchronous with increased streamflow in the Big Lost River below the Moore Diversion (streamgage 13132100), and the resumption of streamflow through the Big Lost River near Arco (streamgage 13132373) and south of Arco (streamgage 13132500) (fig. 18). This response suggests that potentiometric surface altitudes at depths of as much as 60 ft can be rapidly influenced by the resumption of streamflow in the Big Lost River. Thus, although the well cluster data are limited in temporal scale, they support existing understandings of vertical gradients in shallow groundwater (less than 60 ft) in the Big Lost River Valley.
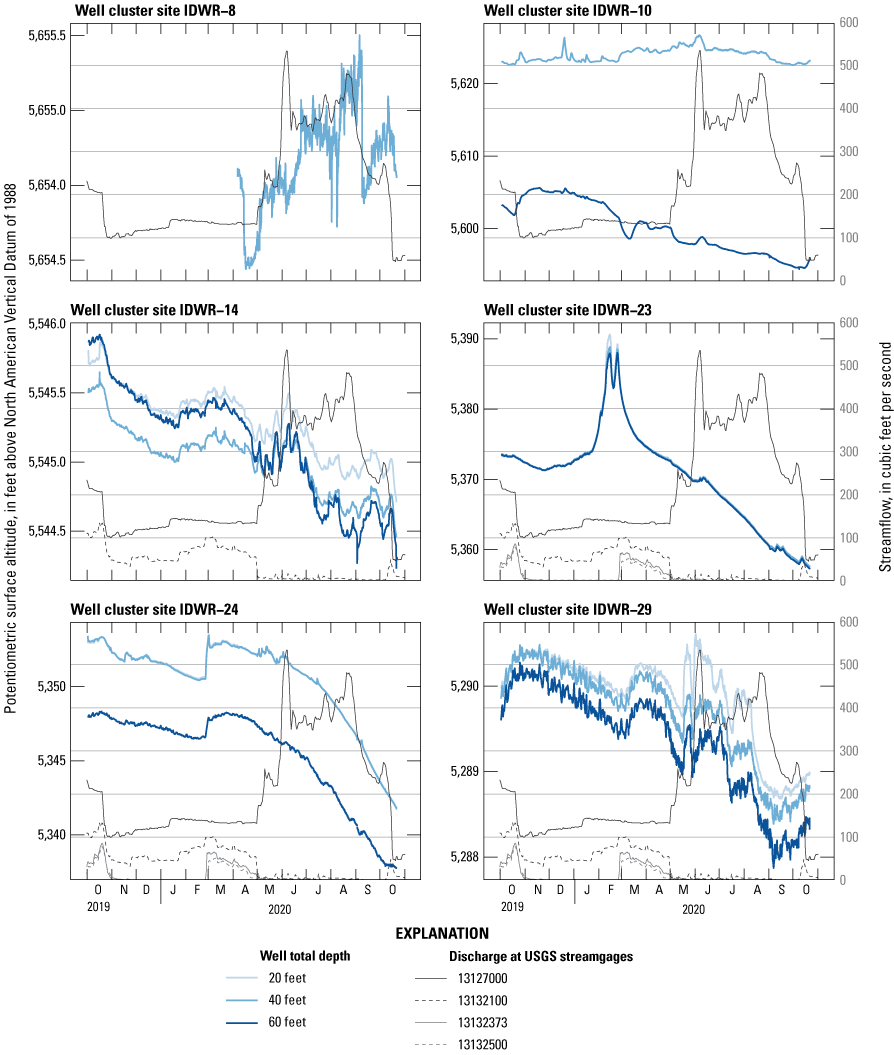
Groundwater potentiometric surface altitudes in well clusters and Big Lost River streamflow at select streamgages in the Big Lost River Valley, south-central Idaho, October 2019–October 2020. Vertical scale varies by an order of magnitude between plots. USGS, U.S. Geological Survey.
Deeper vertical gradients (greater than 60 ft) and connectivity of water-bearing zones in the southern end of the Big Lost River Valley cannot be fully described with existing data. Wells in this part of the basin are variable in depth (fig. 13) and open to Quaternary unconsolidated sediments and Quaternary basalt rocks hydrogeologic units (figs. 11C, 12), but it is unclear if the multiple water-bearing zones are hydraulically connected. Detailed logging for the deep (760-ft) groundwater-level monitoring well, IDWR-22, noted five discrete water-bearing zones and a “cavernous” basalt that “would take more than 500 [gallons per minute] without difficulty” (Crosthwaite and others, 1970a, p. 74). This well is screened below the basalt and open to Quaternary unconsolidated sediments at 656–661 ft (altitude 4,734–4,729 ft) and 685–690 ft (altitude 4,705–4,700 ft). The potentiometric surface altitude (around 4,800 ft) in well IDWR-22 is lower than the altitude in nearby wells (IDWR-21 around 5,400 ft and IDWR-25 around 5,300 ft, fig. 19; and IDWR-23 around 5,370 ft and IDWR-24 around 5,350 ft, fig. 18). Hydrographs of the potentiometric surface altitude over the last 20 years show similar multiyear fluctuations in the deep well (IDWR-22) and the shallower wells (IDWR-21 and IDWR-25) but the shallow wells show more annual variability (fig. 19). This suggests that both shallow and deep water-bearing zones are influenced by similar long-term impacts such as climate variability but does not resolve local hydraulic connection, particularly given the water-taking basalt flow between the water-bearing zones. Doing an aquifer test in a well screened within 100–200 ft of the land surface near Arco and measuring the response in the deep well (IDWR-22) and nearby well clusters (IDWR-23 and IDWR-24) could help to determine hydraulic connection between the shallow (less than 60 ft), middle (100–200 ft) and deep (760 ft) water-bearing zones.
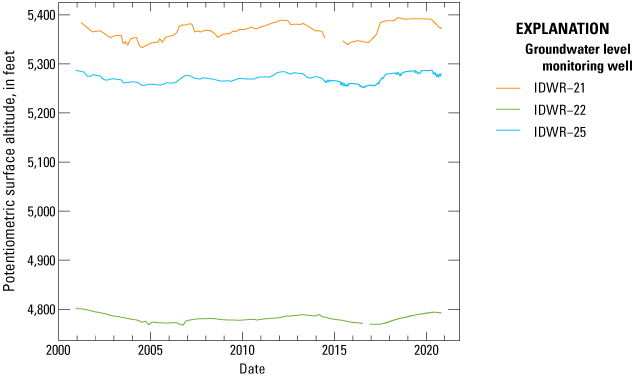
Groundwater potentiometric surface altitude for select wells, Big Lost River Valley, south-central Idaho, 2000–20. Vertical datum could not be verified for all well altitudes.
Insights and Data Gaps
This hydrogeologic framework provides an updated conceptual model and a data-derived three-dimensional hydrogeologic framework model of the hydrogeologic units in the Big Lost River Basin. Combined with existing groundwater-level and streamflow data, the three-dimensional model also provides a means to test hypotheses regarding confining layers, vertical gradients, and controls on groundwater-surface-water interactions, and to address current management questions on water occurrence and movement in the basin. This section addresses some of the water-management insights from and data gaps in the hydrogeologic framework.
Hydrogeologic Controls on Groundwater and Surface Water
The three-dimensional hydrogeologic framework model shows general patterns in subsurface hydrogeologic units and subunits that are consistent with the geologic history and support some previous hypotheses regarding controls on gaining and losing river reaches and the extent of confining subunits. Coarse-grained, highly transmissive subunits occur in the subsurface along the main axis of the valley, and coarse-grained subunits occur on major tributary alluvial fans such as Alder Creek (fig. 8C) and Antelope Creek (fig. 6B), and near Chilly where the Big Lost River enters the main valley (fig. 6A). This pattern is consistent with well-sorted and fluvially reworked sediments expected proximal to the Big Lost River and major tributaries. In contrast, more clay subunits and confining conditions occur at the margins of the valleys, consistent with the occurrence of alluvial fans with more poorly sorted sediment and distal deposition of finer-grained fluvial sediment. Groundwater-level monitoring wells between Darlington and Arco (IDWR-11 to IDWR-21) show the greatest fluctuations in groundwater levels (fig. 15), indicating that highly transmissive subunits in this part of the Big Lost River Valley are susceptible to variations in recharge and groundwater use, and suggesting that declining groundwater levels in this part of the valley could affect streamflows (Dudunake and Zinsser, 2021). These findings are consistent with observations of previous researchers (Crosthwaite and others, 1970a; Johnson and others, 1991; Bassick and Jones, 1992), but the three-dimensional hydrogeologic framework model provides a spatial interpretation of the hydrogeologic units that can be used in the development of a potential future groundwater model.
The three-dimensional hydrogeologic framework model also provides insights into patterns of surface-water losses to and gains from groundwater in the Big Lost River. Previous researchers attributed these patterns to changes in aquifer width proportionate to valley width (Livingston, 1931; Stearns and others, 1938; Crosthwaite and others, 1970a), changes in porosity (Livingston, 1931; Crosthwaite and others, 1970b; Rice and Boyd, 2008), and changes in water quantity (Crosthwaite and others, 1970a). The thickness of the Quaternary unconsolidated sediments is critical for understanding spatial variations in aquifer volume but remains poorly defined. Thicknesses interpreted from geophysical surveys range from about 100 ft near Mackay to 5,000 ft between Mackay and Leslie, and average around 2,000 ft (Crosthwaite and others, 1970a), whereas changes in valley width are on the order of several miles. Thus, changes in valley width likely exert primary control on the volume of the Quaternary unconsolidated sediments and subsequently affect river gains and losses, although depth may be more important to aquifer geometry in the narrow valley near Mackay and fault structures may also play a role throughout the basin.
Historical Big Lost River streamflow losses and gains are associated with changes in the alluvial aquifer geometry and porosity. Losses in the Chilly sinks occur in coarse-grained sediments (fig. 6A) where the valley widens as the upper Big Lost River flows into the main valley. Streamflow gains upstream and downstream from Mackay Reservoir occur in a relatively narrow part of the valley with shallow bedrock (fig. 8B). Losses in the Darlington Sinks occur in a wide part of the valley where the aquifer is coarse-grained (figs. 8A, 9A). Structural controls in this fault-bounded basin also may play a role in aquifer geometry and streamflow losses but could not be thoroughly explored in this study. Streamflow gains occur as the valley narrows upstream from the Moore Diversion and near Arco (fig. 1), but other factors also likely influence streamflow in these areas.
Although the valley narrows near the Moore Diversion, Antelope Creek also enters the Big Lost River Valley in this area. Antelope Creek is diverted for irrigation use and lost to the subsurface (Crosthwaite and others, 1970a; Clark, 2022), thereby contributing to aquifer recharge. Potentiometric surface altitudes also are influenced by interannual variations in precipitation and seasonal irrigation demands (figs. 15–16). High potentiometric surface altitudes are associated with large surface-water gains and low potentiometric surface altitudes are associated with surface-water losses (Dudunake and Zinsser, 2021). Thus, aquifer geometry, groundwater recharge by Antelope Creek, and variations in groundwater use all likely affect the occurrence and magnitude of surface-water gains in the Big Lost River above the Moore Diversion.
Historical streamflow gains in the Big Lost River near Arco likely are influenced by local porosity, aquifer zonation, and changing irrigation practices. Historical accounts described a shallow water formation that fluctuated with the river level (Stearns and others, 1938); more recently, Owsley (2013) reported that the timing of streamflow gains coincided with irrigation season. The three-dimensional hydrogeologic framework model shows a laterally continuous clay subunit in the shallow subsurface (about 50–100 ft below the surface) near Arco (figs. 10, 11C), and shallow (20-ft to 60-ft) wells at well cluster site IDWR-24 show a rapid response to changes in surface-water management (that is, resumption of flows in the Big Lost River through Arco; fig. 18). This response suggests that the application of irrigation water and leakage from the Big Lost River and canals can cause rapid recharge of the alluvial aquifer above the confining layer near Arco, in turn producing streamflow gains downstream in the Big Lost River. Diminishment of streamflow gains over time may be the result of decreased potentiometric surface altitude in the uppermost unconfined zone although the hydraulic connection between this and the underlying confined zone is unclear. If the upper zone is perched, decreased groundwater levels could be influenced primarily by localized effects like decreased incidental recharge (Johnson and others, 1991) due to increased efficiency of irrigation methods (such as canal lining and conversion of flood irrigation to center pivots). If the upper zone is hydraulically connected to the underlying zone, decreased potentiometric altitudes could be affected by decreases in local incidental recharge and regional changes in water use and climate that have caused statistically significant groundwater-level declines throughout the Big Lost River Basin (Sukow, 2017). Given the spatial heterogeneity of subunits within the Quaternary unconsolidated sediments, the upper unconfined zone and lower confined zone likely are hydraulically connected at least at the valley scale. Continued monitoring of groundwater levels in IDWR-24 (in the upper unconfined zone) and IDWR-25 (in the lower confined zone) and (or) doing an aquifer test in IDWR-25 would improve understanding of hydraulic connectivity.
Data Gaps
One of the major limitations of this hydrogeologic framework is that wide spacing between long-term groundwater monitoring wells limits detailed interpretation of the potentiometric surface. A synoptic groundwater-level measurement event that captured upwards of 200 wells, similar to the networks in Crosthwaite and others (1970a) and Bassick and Jones (1992), would provide a clearer understanding of current potentiometric surface altitude and flow directions and potentially would illustrate changes since the 1968 and 1991 measurement events. Likewise, additional data collection at the well clusters would continue to yield insights about interannual and seasonal variability in vertical groundwater gradients. Aquifer tests near Arco could improve understanding of hydraulic connectivity between the shallow (60-ft), middle (100–200-ft) and deep (700-ft) water-bearing zones. Continued monitoring and trend analysis of groundwater levels in irrigated and non-irrigated areas would help in understanding whether declines in groundwater levels are continuing, and if so, whether declines are driven by either climate or water use, or both. In the three-dimensional hydrogeologic framework model, an improved geologic representation that incorporates bedrock contacts, geometry, and faults would improve the understanding of recharge at the valley margins. Additional geophysical work to refine the Quaternary unconsolidated sediments unit geometry could help define aquifer volumes.
Summary
Water resources in the Big Lost River Basin of south-central Idaho are vital to local irrigated agriculture, ranching, domestic, and other uses, and also contribute recharge to the Eastern Snake River Plain aquifer, but the last comprehensive study of water resources in the basin was completed over fifty years ago. The U.S. Geological Survey, in cooperation with the Idaho Department of Water Resources, developed an updated hydrogeologic framework of the Big Lost River Basin to help address current challenges in water-resources management.
The hydrogeologic framework of the Big Lost River Basin provides a conceptual basis for understanding groundwater resources in the basin and comprises three main parts: (1) a conceptual description of hydrogeologic units, (2) a three-dimensional hydrogeologic framework model used as a tool to represent the spatial occurrence of the hydrogeologic units, and (3) a description of groundwater occurrence and movement within the hydrogeologic units. The Lost River Basin has four main hydrogeologic units. First, the Paleozoic sedimentary rocks unit, comprising carbonate rocks with lesser siliciclastic rocks, forms the major bedrock aquifer and contributes subsurface recharge at the valley margins to the Quaternary unconsolidated sediments unit. Second, the Tertiary volcanic rocks unit (primarily andesites, dacites, and tuffs) is a locally important source of water, particularly in faulted and fractured zones. Third, the Quaternary basalt rocks unit comprises several important water-bearing zones in the southern end of the Big Lost River Valley. Finally, the most important hydrogeologic unit and main source of groundwater in the Big Lost River Basin is the Quaternary unconsolidated sediments unit. This unit comprises the alluvial aquifer and is further subdivided by grain size or other distinguishing characteristics into six subunits: boulders, gravel, sand, clay, cemented sediments, and soil.
The three-dimensional hydrogeologic framework model describes the spatial distribution of the hydrogeologic units and provides insights into hydrogeologic controls on long-observed patterns of water movement. Within the Quaternary unconsolidated sediments unit, coarse and well-sorted subunits (gravel and sand) generally occur in the center of the valley and on major tributary fans (Alder and Antelope Creeks), and spatially discontinuous and fine subunits (clays) are located along the valley margins. This general pattern results in some local confinement at valley margins, and greater horizontal hydraulic conductivity in the center of the valley than along the sides of the valley. Historically losing reaches of the Big Lost River in the Chilly and Darlington Sinks are associated with valley widening and coarse unconsolidated sediment subunits (sand and gravel). Historically gaining reaches of the Big Lost River are associated with valley narrowing (above and below the Mackay Reservoir, above the Moore Diversion and near Arco), recharge from surface water and irrigation (above the Moore Diversion and near Arco), and confining layers (near Arco) in the Quaternary unconsolidated sediments hydrogeologic unit.
Groundwater flow generally mimics topography and potentiometric surface altitudes show substantial seasonal, interannual, and decadal fluctuations in response to annual precipitation, irrigation, recharge and climate variability; these fluctuations likely affect streamflow in the Big Lost River. Data on vertical gradients are limited, but downward gradients generally spatially align with areas of historical streamflow losses and shallow groundwater recharge. Well opening data captured in the hydrogeologic framework well database provide insights into water-bearing zones, including general patterns in the depth of water-bearing zones and the occurrence of multiple water-bearing zones in the southern end of the Big Lost River Valley, although the vertical hydraulic connection between the southern zones is unclear. Overall, the hydrogeologic framework provides a conceptual basis to understand groundwater resources in the Big Lost River Basin and largely aligns with previous assessments of the hydrogeology. The use of the three-dimensional hydrogeologic framework model provides a three-dimensional representation of the hydrogeologic units that, paired with water data, yields new insights to help inform current water-resources management in the basin.
References Cited
Ackerman, D.J., Rattray, G.W., Rousseau, J.P., Davis, L.C., and Orr, B.R., 2006, A conceptual model of ground-water flow in the eastern Snake River Plain aquifer at the Idaho National Laboratory and vicinity with implications for contaminant transport: U.S. Geological Survey Scientific Investigations Report 2006–5122, 62 p., accessed July 6, 2020, at https://doi.org/10.3133/sir20065122.
Ackerman, D.J., Rousseau, J.P., Rattray, G.W., and Fisher, J.C., 2010, Steady-state and transient models of groundwater flow and advective transport, Eastern Snake River Plain aquifer, Idaho National Laboratory and vicinity, Idaho: U. S. Geological Survey Scientific Investigations Report 2010–5123, 220 p., accessed April 18, 2018, at https://doi.org/10.3133/sir20105123.
Bassick, M.D., and Jones, M.L., 1992, Aquifer-test results, direction of ground-water flow, and 1984–90 annual ground-water pumpage for irrigation, lower Big Lost River Valley, Idaho: U.S. Geological Survey Water-Resources Investigations Report 92–4006. 1 pl., scale 1:100,000, accessed April 18, 2018, at https://doi.org/10.3133/wri924006.
Bernal, R., 2016, Petition to designate a critical groundwater area in the Big Lost River Basin: Butte County, Idaho: Submission to the Idaho Department of Water Resources, docket #P-CGWA-2016-001, 17 p., accessed August 24, 2018, at https://idwr.idaho.gov/files/legal/P-CGWA-2016-001/P-CGWA-2016-001-20160919-Petition-to-designate-the-Big-Lost-River-Basin-as-a-CGWA.pdf.
Bernal, R., and Broadie, M., 2017, Notice of withdrawal of petitions—Butte County, ID: Submission to the Idaho Department of Water Resources, docket #P-CGWA-2016-001, 3 p., accessed February 4, 2021, at https://idwr.idaho.gov/files/legal/P-CGWA-2016-001/P-CGWA-2016-001-20170620-Notice-of-Withdrawal-of-Petitions.pdf.
Broadie, M., 2017a, Designation of Big Lost River Basin as a ground water management area (GWMA): Butte County, Idaho, Submission to the Idaho Department of Water Resources, docket #P-CGWA-2016-001, 2 p., accessed February 4, 2021, at https://idwr.idaho.gov/files/legal/P-CGWA-2016-001/P-CGWA-2016-001-20170109-Broadie-Letter-re-designation-of-Big-Lost-River-Basin-GWMA.pdf.
Broadie, M., 2017b, Response to petition to create a critical ground water area in the Big Lost River basin: Butte County, Idaho, Submission to the Idaho Department of Water Resources, docket #P-CGWA-2016-001. 2 p., accessed February 4, 2021, at https://idwr.idaho.gov/files/legal/P-CGWA-2016-001/P-CGWA-2016-001-20170123-Response-to-Petition-to-Create-a-CGWA-in-the-Big-Lost-River-Basin.pdf.
Clark, A., 2022, Groundwater budgets for the Big Lost River Basin, south-central Idaho, 2000–19, chap. C of Zinsser, L.M., ed., Characterization of water resources in the Big Lost River Basin, south-central Idaho: U.S. Geological Survey Scientific Investigations Report 2021–5078–C, 111 p., https://doi.org/10.3133/sir20215078C
Crosthwaite, E.G., Thomas, C.A., and Dyer, K.L., 1970b, Considerations for water use and management in the Big Lost River Basin, Idaho—A supplemental report: U. S. Geological Survey Open-File Report 70–92, 32 p., https://doi.org/10.3133/ofr7092.
Crosthwaite, E.G., Thomas, C.A., and Dyer, K.L., 1970a, Water resources in the Big Lost River Basin, south-central Idaho: U.S. Geological Survey Open-File Report 70–93, 109 p., accessed April 19, 2018, at https://doi.org/10.3133/ofr7093.
Dudunake, T.J., and Zinsser, L.M., 2021, Surface-water and groundwater interactions in the Big Lost River Valley, south-central Idaho, chap. B of Characterization of water resources in the Big Lost River Basin, south-central Idaho: U.S. Geological Survey Scientific Investigations Report 2021–5078–B, 33 p., https://doi.org/10.3133/sir20215078B.
Hodges, M.K.V., Link, P.K., and Fanning, C.M., 2009, The Pliocene Lost River found to west—Detrital zircon evidence of drainage disruption along a subsiding hotspot track: Journal of Volcanology and Geothermal Research, v. 188, nos. 1–3, p. 237–249. [Also available at https://doi.org/10.1016/j.jvolgeores.2009.08.019.]
Idaho Department of Water Resources, 2013, Enhanced Snake Plain Aquifer Model Version 2.1—Final report: Idaho Department of Water Resources, 91 p., accessed April 6, 2021, at https://idwr.idaho.gov/files/projects/espam/browse/ESPAM_2_Final_Report/ESPAM21FinalReport.pdf.
Idaho Department of Water Resources, 2018, Wells: Idaho Department of Water Resources GIS dataset, accessed March 19, 2019, at https://data-idwr.opendata.arcgis.com/datasets/wells.
Idaho Department of Water Resources, 2021b, Groundwater level data: Idaho Department of Water Resources map and GIS data hub, accessed September 10, 2021, at https://data-idwr.opendata.arcgis.com/ and https://idwr.idaho.gov/water-data/groundwater-levels.
Idaho Department of Water Resources, 2021a, Well construction search: Idaho Department of Water Resources database, accessed February 3, 2021, at https://idwr.idaho.gov/Apps/appsWell/WCInfoSearchExternal/.
Kenworthy, M.K., Rittenour, T.M., Pierce, J.L., Sutfin, N.A., and Sharp, W.D., 2014, Luminescence dating without sand lenses—An application of OSL to coarse-grained alluvial fan deposits of the Lost River Range, Idaho, USA: Quaternary Geochronology, v. 23, p. 9–25. [Also available at https://dx.doi.org/https://doi.org/10.1016/j.quageo.2014.03.004.]
Kuntz, M.A., Covington, H.R., Schorr, L.J., Link, P.K., Kuntz, M.A., and Piatt, L.B., 1992, An overview of basaltic volcanism of the eastern Snake River Plain, Idaho, chap. 12 of Link, P.K., Kuntz, M.A., and Piatt, L.B., eds., Regional geology of eastern Idaho and western Wyoming: Boulder, Colorado, Geological Society of America, p. 227–267. [Also available at https://doi.org/10.1130/MEM179-p227.]
Lewis, R.S., Link, P.K., Stanford, L.R., and Long, S.P., 2012, Geologic map of Idaho: Moscow, University of Idaho, Idaho Geological Survey Geologic Maps M-9, 978-1-55765-118-1. 1 pl., scale 1:750,000, accessed October 10, 2020, at https://www.idahogeology.org/product/m-9.
Link, P.K., and Janecke, S.U., 1999, Geology of east-central Idaho—Geologic roadlogs for the Big and Little Lost River, Lemhi, and Salmon River Valleys, in Hughes, S.S., and Thackray, G.D., eds., Guidebook to the geology of eastern Idaho: Pocatello, Idaho, Idaho Museum of Natural History, p. 295–334. [Also available at https://digitalatlas.cose.isu.edu/geo/gsa/papers/gsac5p18.pdf.]
Moye, F.J., Hackett, W.R., Blakley, J.D., and Snider, L.G., 1988, Regional geologic setting and volcanic stratigraphy of the Challis volcanic field, central Idaho, in Link, P.K., and Hackett, W.R., eds., Guidebook to the geology of central and southern Idaho: Moscow, Idaho Geological Survey Bulletin, p. 87–97. [Also available at http://citeseerx.ist.psu.edu/viewdoc/download?doi=10.1.1.523.1239&rep=rep1&type=pdf.]
Natural Resources Conservation Service, 2017, Idaho water supply outlook report, May 1, 2017: Natural Resources Conservation Service, 32 p., accessed March 14, 2021, at https://www.nrcs.usda.gov/wps/portal/nrcs/detailfull/id/snow/waterproducts/?cid=stelprdb1241057
Natural Resources Conservation Service, 2020, Idaho water supply outlook report, May 1, 2020: Natural Resources Conservation Service, 27 p., accessed March 14, 2021, at https://www.nrcs.usda.gov/wps/portal/nrcs/detailfull/id/snow/waterproducts/?cid=stelprdb1241057.
Owsley, D., 2013, Application for transfer no. 77610 in the name of Parkinson Farms: Boise, Idaho Department of Water Resources Memo, 18 p., accessed June 1, 2020, at https://idwr.idaho.gov/apps/ExtSearch/RelatedDocsTransfers.asp?TransferNumber=77610.
Patterson, S.J., 2006, Sedimentology and geomorphology of Quaternary alluvial fans with implications to growth strata, Lost River Range: Bozeman, Montana State University, M.S. thesis in Earth Sciences, 161 p., accessed October 7, 2020, at https://scholarworks.montana.edu/xmlui/handle/1/2025.
Pierce, K.L., and Morgan, L.A., 1992, The track of the Yellowstone hot spot—Volcanism, faulting and uplift, chap. 1 of Link, P.K., Kuntz, M.A., and Platt, L.B., eds., Regional geology of eastern Idaho and western Wyoming: Boulder, Colorado, Geological Society of America Memoir 179, p. 1–53., https://doi.org/10.1130/MEM179-p1.
Pierce, K.L., and Scott, W.E., 1982, Pleistocene episodes of alluvial-gravel deposition, southeastern Idaho, in Bonnichsen, B., and Breckenridge, R.M., eds., Cenozoic geology of Idaho:Moscow, Idaho Bureau of Mines and Geology Bulletin 26, p. 685–702. [Also available at https://www.researchgate.net/publication/265751313_Pleistocene_Episodes_of_Alluvial-Gravel_Deposition_Southeastern_Idaho.]
Skipp, B., Hoggan, R.D., Schleicher, D.L., and Douglass, R.C., 1979, Upper Paleozoic carbonate bank in east-central Idaho; Snaky Canyon, Bluebird Mountain, and Arco Hills formations, and their paleotectonic significance: U.S. Geological Survey Bulletin 1486, 78 p., accessed March 10, 2021, at https://doi.org/10.3133/b1486.
Skipp, B., Snider, L., Janecke, S., and Kuntz, M., 2009, Geologic map of the Arco 30 × 60 minute quadrangle, south-central Idaho: Moscow, Idaho Geological Survey Geologic Maps GM-47, 1 pl., scale 1:100,000, accessed October 2, 2020, at https://www.idahogeology.org/product/gm-47.
Stearns, H.T., Crandall, L., and Steward, W.G., 1938, Geology and ground-water resources of the Snake River Plain in southeastern Idaho: U.S. Geological Survey Water Supply Paper 774. 268 p., 6 pls., accessed November 5, 2018, at https://doi.org/10.3133/wsp774.
Stein, R.S., and Bucknam, R.C., 1985, Proceedings of workshop XXVIII on the Borah Peak, Idaho earthquake: U.S. Geological Survey Open-File Report 85–290A, 704 p., accessed November 7, 2018, at https://doi.org/10.3133/ofr85290A.
Sukow, J., 2017, Groundwater in the Big Lost River Valley: Boise, Idaho Department of Water Resources Memo, 37 p, accessed November 5, 2018, at https://idwr.idaho.gov/legal-actions/administrative-actions/big-lost-river-basin-CGWA-petition.html.
U.S. Geological Survey, 2016, The StreamStats program for Idaho: U.S. Geological Survey web page, accessed February 8, 2021, at https://water.usgs.gov/osw/streamstats/idaho.html.
U. S. Geological Survey, 2018, Divisions of geologic time—Major chronostratigraphic and geochronologic units: U.S. Geological Survey Fact Sheet 2018–3054, accessed March 8, 2021, at https://doi.org/10.3133/fs20183054.
U.S. Geological Survey, 2020, The national map, 3DEP elevation index, DEM products: U.S. Geological Survey web page, accessed April 15, 2020, at https://apps.nationalmap.gov/viewer/.
U.S. Geological Survey, 2021a, National Water Information System—Web interface: U.S. Geological Survey database, accessed June 1, 2021, at https://nwis.waterdata.usgs.gov/nwis and https://doi.org/10.5066/F7P55KJN.
U.S. Geological Survey, 2021b, USGS GeoLog locator: U.S. Geological Survey web page, accessed January 29, 2021, at https://webapps.usgs.gov/GeoLogLocator/#!/ and https://doi.org/10.5066/F7X63KT0.
Welhan, J.A., Clemo, T.M., and Gégo, E.L., 2002, Stochastic simulation of aquifer heterogeneity in a layered basalt aquifer system, eastern Snake River Plain, Idaho, in Link, P.K., and Mink, L.L., eds., Geology, hydrogeology, and environmental remediation—Idaho National Engineering and Environmental Laboratory, eastern Snake River Plain, Idaho: Boulder, Colorado, Geological Society of America, p. 225–247., https://doi.org/10.1130/0-8137-2353-1.225.
Whitehead, R.L., 1992, Geohydrologic framework of the Snake River plain regional aquifer system, Idaho and eastern Oregon: U.S. Geological Survey Professional Paper 1408-B. 39 p., accessed March 9, 2021, at https://doi.org/10.3133/pp1408B.
Wood, S.H., Wurts, C., Lane, T., Ballenger, N., Shaleen, M., and Totorica, D., 1985, The Borah Peak, Idaho earthquake of October 28, 1983—Hydrologic effects: Earthquake Spectra, v. 2, no. 1, p. 127–150, https://doi.org/10.1193/1.1585305.
Zinsser, L.M., 2021, Hydrogeologic framework of the Big Lost River Basin, south-central Idaho—Hydrogeologic framework model and well data: U.S. Geological Survey data release, https://doi.org/10.5066/P911S9LF.
Appendix 1. Borehole Geophysical Methods and Logs
Borehole Geophysical Methods
Borehole geophysical data and borehole videos were collected from 10 groundwater-level monitoring wells that ranged in depth from 143 to 760 feet below land surface. Borehole video files were used to confirm well depth, well casing integrity, well casing size, and well obstructions. Geophysical logs were collected only in cased borehole sections because of the concerns of neutron and gamma-gamma logging tools being ensnared in uncased (open) wells. Borehole geophysical logs were used to examine lithology and approximate location and thickness of sediment and rock units. Well-driller reports, when available, were used initially to identify general borehole lithology for each well. However, this information was general or incomplete so geophysical logs were used to update lithology by estimating the location of unconsolidated sediment and rock contacts and changes in unconsolidated sediment layering where reasonable. Where well-driller reports were not available, well lithology was approximated based on well-driller reports for nearby wells (2 miles away or less) and geophysical data. Wells without well-driller reports presented a higher degree of uncertainty than wells with well-driller reports when interpreting lithology. Large borehole diameters, unknown construction materials, and use of multiple casing strings also present more geophysical uncertainty observed through muted signal response. Because of the limitations in interpretation, a generalized lithology approach was applied for each of the 10 wells reviewed as part of this study. Sediment layers were described as clay, silt, sand, and gravel layers or as a combination of multiple grain sizes such as gravel with clay, gravel with sand, and sand with clay. Sedimentary rock lithologies consisted of sandstone and limestone; volcanic rock lithologies consisted of basalt.
Natural Gamma Logs
Natural gamma logs record gamma radiation emitted by naturally occurring radioisotopes. The natural gamma detector measures total gamma radiation without distinguishing among individual contributions of the various isotopes. The natural gamma signal was used to identify changes in lithologies by examining signal changes. For example, natural gamma was used to identify a transition from a clay layer to a gravel layer or from unconsolidated sediments to basalt rock by examining subtle signal changes. Natural gamma logs are especially useful when used in combination with well-driller reports because continuous geophysical logs are well suited to approximate where changes to lithologic contacts occur by indicating where the signal changes, whereas well-driller reports can only approximate the location of contacts based on cutting returns. For this study, the natural gamma log was used to estimate the well lithology in combination with available well-driller reports.
Neutron Logs
Neutron logs are a general indicator of hydrogen content, and when combined with natural gamma logs (which indicate sediment location), they can be used to identify perched groundwater. The neutron detector continuously records induced radiation produced by bombarding surrounding media (casing, formation, and fluid) with fast neutrons (energies greater than 105 electron volts) from a sealed neutron source, which collide with surrounding atomic nuclei until they are captured (Keys, 1990). The neutron probe used by the USGS INL Project Office has an americium/beryllium neutron source and a helium-3 detector that counts slow (thermal) neutrons (those that have energies less than 0.025 electron volts). The neutron logs were collected through drill casing after confirming casing integrity using borehole videos. For this study, the neutron log and approximated porosity log were used to estimate where groundwater was likely moving and where porosity was elevated. The neutron log also was used in combination with the natural gamma log to identify changes in lithology.
Gamma-Gamma Dual Density Logs
Gamma-gamma dual density logging detects Compton-scattered gamma rays that originate from a fully encapsulated 0.2-curie cesium-137 source. The radioactive source is threaded into the bottom of the tool during operation, like the neutron source. The intensity of the gamma radiation reflected back to the probe is a function of the media’s electron density after it is backscattered or absorbed in a drill hole, borehole fluid, or surrounding media. In this study, an omni-directional, dual detector sonde that responds to density variation in counts per second was used; it registered higher counts per second for lower density material. The gamma-gamma dual density log was used to examine bulk density changes in the rock and sediment layers.
Fluid Logs
Fluid specific conductance and temperature were measured in 3 of the 10 wells in this study; however, the logs were run under partially disturbed fluid conditions where borehole videos were run as the first log. Specific conductance measures the ability of groundwater to conduct electric current and changes in specific conductance generally are related to dissolved solid concentrations within the fluid column. Specific conductance provides a general indicator for changing water chemistry when run in a vertical fluid column. Water temperature also can provide evidence for changes in water chemistry and (or) suggest where groundwater is moving or not moving within a vertical borehole. For this study, fluid logs provided a good indicator for where groundwater flow occurred within a well column and the general range of specific conductance and water temperature for the well.
Borehole Geophysical Logs
The geophysical logs for 10 groundwater-level monitoring wells in the Big Lost River Basin are available in an Adobe Acrobat® PDF file for download at https://doi.org/10.3133/sir20215078A.
References Cited
Keys, W.S., 1990, Borehole geophysics applied to ground-water investigations: U.S. Geological Survey Techniques of Water-Resources Investigations 02-E2, 150 p., accessed March 13, 2021, at https://doi.org/10.3133/twri02E2.
U.S. Geological Survey, 2021b, USGS GeoLog locator: U.S. Geological Survey web page, accessed January 29, 2021, at https://webapps.usgs.gov/GeoLogLocator/#!/ and https://doi.org/10.5066/F7X63KT0.
Conversion Factors
U.S. customary units to International System of Units
Datums
Vertical coordinate information, where known, is referenced to the North American Vertical Datum of 1988 (NAVD 88).
Horizontal coordinate information is referenced to the North American Datum of 1983 (NAD 83).
Altitude, as used in this report, refers to distance above the vertical datum (where known).
Supplemental Information
Specific conductance is given in microsiemens per centimeter at 25 degrees Celsius (µS/cm at 25 °C).
Publishing support provided by the U.S. Geological Survey
Science Publishing Network, Tacoma Publishing Service Center
For more information concerning the research in this report, contact the
Director, Idaho Water Science Center
U.S. Geological Survey
230 Collins Road
Boise, Idaho 83702-4520
Suggested Citation
Zinsser, L.M., 2021, Hydrogeologic framework of the Big Lost River Basin, south-central Idaho, chap. A of Zinsser, L.M., ed., Characterization of water resources in the Big Lost River Basin, south-central Idaho: U.S. Geological Survey Scientific Investigations Report 2021–5078–A, 42 p., https://doi.org/10.3133/sir20215078A.
ISSN: 2328-0328 (online)
Study Area
Publication type | Report |
---|---|
Publication Subtype | USGS Numbered Series |
Title | Hydrogeologic framework of the Big Lost River Basin, south-central Idaho, chap. A of Zinsser, L.M., ed., Characterization of water resources in the Big Lost River Basin, south-central Idaho |
Series title | Scientific Investigations Report |
Series number | 2021-5078 |
Chapter | A |
DOI | 10.3133/sir20215078A |
Year Published | 2021 |
Language | English |
Publisher | U.S. Geological Survey |
Publisher location | Reston, VA |
Contributing office(s) | Idaho Water Science Center |
Description | Report: viii, 42 p.; Appendix; Data Release |
Country | United States |
State | Idaho |
Other Geospatial | Big Lost River Basin |
Online Only (Y/N) | Y |
Additional Online Files (Y/N) | Y |
Google Analytic Metrics | Metrics page |