Integrated Science for the Study of Microplastics in the Environment—A Strategic Science Vision for the U.S. Geological Survey
Links
- Document: Report (16.8 MB pdf) , HTML , XML
- Download citation as: RIS | Dublin Core
Executive Summary
Overview
Evidence of the widespread occurrence of microplastics throughout our environment and exposure to humans and other organisms over the past decade has led to questions about the possibility of health hazards and mitigation of exposures. This document discusses nanoplastics as well as microplastics (referred to solely as microplastics); the microplastics have a range from 1 micrometer to 5 millimeters (1 μm–5 mm) in length, whereas the nanoplastics are less than 1 μm in length (sidebar ES1).
A myriad of environmental exposure pathways with microplastics to humans and wildlife, including ingestion, inhalation, and bodily absorption, are likely to exist. A growing body of evidence has documented bioaccumulation of microplastics in tissues and organs of humans and wildlife, benthic community effects, and potential nutritional and reproductive effects in some wildlife species. Understanding if or when environmental exposures pose a health risk is complicated by the diversity of microplastic sizes, morphologies, polymer types, and chemicals added during manufacturing or sorbed from the environment; ongoing challenges in analytical methods used to detect, quantify, and characterize microplastics and associated chemicals in our ecosystems; and the fact that ecotoxicological studies regarding microplastics are still in their infancy. Therefore, the study of environmental exposures and potential related health hazards of microplastics to the public and wildlife is a One Health (sidebar ES2) research topic that necessitates integrated science approaches.
A better understanding of the sources, pathways, fate, and biological effects of microplastics has become a priority of the Federal Government, State governments, Tribes, stakeholders, and the public. Examples of Federal and State microplastic-focused legislation and programs to prioritize microplastic research and reduction include the Federal Microbead-Free Waters Act of 2015, California Senate Bills 1422 and 1263 (2018), the U.S. Environmental Protection Agency (EPA) Trash Free Waters Program, the National Institute of Standards and Technology’s Microplastic and Nanoplastic Metrology project, and Minnesota’s microplastic project. With its unique expertise and capabilities, the U.S. Geological Survey (USGS) is well positioned to help fill some of the most important microplastic science gaps.
This strategic science vision document for microplastics identifies current (2023) microplastic science gaps and prioritizes research relevant to the mission, expertise, and capabilities of the USGS. It is intended for USGS scientists and stakeholders to use as a starting point for planning, prioritizing, and designing collaborative environmental microplastic science. Many of the microplastic science gaps and priorities are scalable, from local to national, and thus, can be made commensurate with available funding and evolving analytical and field tools, laboratory capacity, and stakeholder needs. Current (2023) or future research by academia and other Federal or State agencies, and Tribes may be aimed at some of the same microplastic science gaps identified in this document. Therefore, this document can be used as an information resource to maximize strengths and capabilities and minimize redundancy in communication and collaboration.
Sidebar ES1. Microplastic Terminology
Common terminology for the categorization of plastic debris, modified from Hartmann and others (2019).
Macroplastics
The term “macroplastics” is used to describe plastic fragments (greater than 1 centimeter [cm]) that are created from the fragmentation of plastic as a result of photodegradation and mechanical abrasion due to wind action.
Mesoplastics
The term “mesoplastics” describes plastic fragments such as virgin (newly manufactured) resin particles. They are typically 5–10 millimeters (mm) in length and are created from the breakdown of macroplastics.
Microplastics
Microplastics are defined as smaller plastic particles that are approximately 1 micrometer (μm) to 5 mm in size; these particles in the environment result from the disposal and breakdown of consumer products and industrial waste. There are two types of microplastics: primary and secondary. Some examples include the following:
Existing Capabilities and Laboratory Capacities
USGS capabilities relevant to the microplastic science gaps identified in this document include field and analytical methods development, nationwide stream and groundwater monitoring networks, hydrological testing facilities, and chemistry and toxicology laboratories. Additionally, the USGS has long-standing partnerships with other Federal and State agencies, Tribes, universities, and stakeholder groups. These partnerships jointly pursue research on emerging topics and the development or enhancement of methods and technologies. USGS scientists include hydrologists, chemists, biologists, microbiologists, ecologists, wildlife toxicologists, physical scientists, statisticians, and modelers, among others. With its nationwide network of offices and staff, the USGS is unique in its ability to design and use nationally distributed and nationally consistent field and laboratory protocols with rigorous quality assurance and quality control (QA/QC) which are in line with the baseline requirements of the USGS Quality Management System policy (revised in 2022, U.S. Geological Survey, 2022). In 2023, scientists using laboratory equipment can detect and quantify microplastics in environmental samples. However, standardized sampling protocols, equipment upgrades, and methods development are needed to automate analytical processes to improve efficiency, cost, and quality assurance, to achieve production-level capabilities for analyses. An integrated science approach will advance current understanding of environmental microplastic occurrence, sources, pathways, exposure, biological effects, and mitigation (sidebar ES3).
Sidebar ES2. Definition of One Health Within the Department of the Interior
One Health is a transdisciplinary scientific approach that builds capacity, fosters trust among multidisciplinary partners and stakeholders, and promotes good stewardship of natural and cultural resources with the goal of optimizing human, plant, animal, and ecosystem health.
Scope of Science
This document describes both short- and long-term science opportunities related to the nationally relevant study of microplastics. Short-term opportunities are those that could be achieved in the next 1–2 years. Long-term opportunities, with a timeframe of 3 or more years, are those that require methods development or equipment acquisition, are larger in spatial and temporal scale, build on other science gaps, and cannot be funded with existing resources.
Sidebar ES3. Vision for the U.S. Geological Survey’s Study of Microplastics in the Environment
The broad geographic footprint of U.S. Geological Survey (USGS) science centers across the Nation, multidisciplinary natural science expertise, and nationally consistent laboratory and field capabilities will uniquely position the USGS to support a nationwide integrated science approach to microplastic risks in our environment. This approach can deliver nationally relevant information in support of stakeholder decisions related to microplastics including the identification and data analysis to aid with both mitigation and prevention of microplastic-associated hazards for public and environmental health.
The microplastic science opportunities were identified through literature gap analysis and consideration of USGS expertise and capabilities. Importantly, the USGS capabilities include utilization of long-standing partnerships with other agencies to leverage expertise and funding. The science opportunities that could be addressed by USGS capabilities are organized into six categories: (1) environmental sources, pathways, and fate; (2) human and wildlife exposure routes; (3) ecotoxicology; (4) sampling protocols; (5) analytical methods; and (6) interdisciplinary science at the USGS to support microplastic research (table ES1). These opportunities are described in detail in later sections of this document.
Table ES1.
Short- and long-term science opportunities for researching microplastics that could be addressed by U.S. Geological Survey capabilities.[USGS, U.S. Geological Survey, QA/QC, quality assurance and quality control]
1.0. Introduction
Microplastics are a specific class of plastics characterized by their size, generally defined as plastics smaller than 5 millimeters (mm) but larger than 1 micrometer (μm) (see sidebar 1 for microplastic terminology; Hartmann and others, 2019). The term “microplastic” was first used in scientific literature to describe plastic pollution in 1990 (Ryan and Moloney, 1990). Since then, interest in microplastics has grown considerably, especially in recent years: the number of scientific articles on microplastics each year increased from 4 in 2010 to 3,847 in 2023 (based on a Web of Science search using keyword “microplastics”; Web of Science, 2024). This document is focused on microplastics, but the microplastic science gaps and opportunities identified herein are also applicable to smaller nanoplastics (less than [<] 1 μm).
Sidebar 1. Microplastic Terminology
Common terminology for the categorization of plastic debris, modified from Hartmann and others, 2019.
Macroplastics
The term “macroplastics” is used to describe plastic fragments (greater than 1 centimeter [cm]) that are created from the fragmentation of plastic as a result of photodegradation and mechanical abrasion due to wind action.
Mesoplastics
The term “mesoplastics” describes plastic fragments such as virgin (newly manufactured) resin particles. They are typically 5–10 millimeters (mm) in length and are created from the breakdown of macroplastics.
Microplastics
Microplastics are defined as smaller plastic particles that are approximately 1 micrometer (μm) to 5 mm in size; these particles in the environment result from the disposal and breakdown of consumer products and industrial waste. There are two types of microplastics: primary and secondary. Some examples include the following:
Most microplastic research to date has focused on microplastics in marine waters, in part because of past research on the ocean as the final sink for highly visible and attention-grabbing microplastics and the early focus on the Pacific garbage patch (Ryan, 2015). Estimates of the mass of plastic particles smaller than 5 mm in the world's oceans are as high as 35,000 metric tons (Eriksen and others, 2014). Despite the early focus on the marine environment, recent research has documented that microplastics have been detected in other environmental compartments (physical environments separated by spatial differences), including in freshwaters, urban stormwaters, irrigation waters, seafood, soils, air, and rain (Cole and others, 2011; Li and others, 2018; Smith and others, 2018; Enyoh and others, 2019; Wetherbee and others, 2019; Qi and others, 2020), indicating the myriad environmental pathways of exposure to humans and wildlife. Although the research is still nascent, evidence documents that microplastics are now in human blood (Leslie and others, 2022) and lung tissue (Jenner and others, 2022). A growing body of evidence also documents that microplastics can adversely affect benthic communities (Redondo-Hasselerharm and others, 2020) and have been implicated in adverse effects on nutrition and reproduction in wildlife (Susanti and others, 2020). The principle of One Health describes the interdependence of human, wildlife, and ecosystem health (see sidebar 2; see section 3.0., “Vision for Integrated Science”). There is increasing evidence that microplastics may present a potential One Health hazard.
Sidebar 2. Definition of One Health Within the Department of the Interior
One Health is a transdisciplinary scientific approach that builds capacity, fosters trust among multidisciplinary partners and stakeholders, and promotes good stewardship of natural and cultural resources with the goal of optimizing human, plant, animal, and ecosystem health.
Microplastic research increasingly addresses more complex questions with improvements in field collection and analytical methods. Our understanding of microplastic sources and pathways is improving through more holistic, watershed-scale mass-balance approaches (Fahrenfeld and others, 2019). Research into biological exposure is demonstrating microplastic uptake in wildlife across trophic levels and at different life stages (Athey and others, 2020; Cousin and others, 2020). In addition to monitoring data, laboratory studies are addressing microplastic toxicity, documenting both chemical and physical effects (Huang and others, 2020), including physical interactions among polymers and exposed organisms (digestion blockage and tissue abrasion), and effects from chemical leachates and absorbed contaminants (see sidebar 3 for terminology concerning chemical interactions).
Sidebar 3. Chemical Interaction Terminology
Definition of common chemical interaction terms, modified from a variety of sources (Hartmann and others, 2017; Lambert and Wagner, 2017; Hartmann and others, 2019).
Additive
An additive is a chemical added to a plastic polymer during manufacturing to improve characteristics, such as elasticity, resistance to weathering, or flame retardance and performance.
Desorption
The reverse of sorption is desorption, during which the sorbed chemical is released from the plastic back to the fluid stage.
Plastic polymer
A plastic polymer has a molecular structure consisting primarily of a large number of similar units (monomers) bonded together (that is, synthetic organic materials in plastics and resins). Properties of plastic polymers (surface charge, surface area, molecular chain arrangement, functional groups, acid-base character) affect the sorption of chemicals.
It is difficult to compare studies across all lines of research, in part due to nonstandard analytical, sampling, and experimental methods (Weis, 2020). Intergovernmental agencies, including the World Health Organization, the Organisation for Economic Co-operation and Development, and the Group of 20, have called for prioritizing microplastic research (Marsden and others, 2019; Organisation for Economic Co-operation Development, 2019; Xu and Karlsson, 2019). There is a need for organized research by Federal agencies, such as the USGS, who have the expertise to systematically approach the topic of microplastics. The USGS can fulfill this need by using a consistent methodology and prioritizing research based on identified regulatory needs, monitoring data gaps, applied modeling approaches, and ecotoxicological investigations.
Early local, State, and Federal government regulations concerning microplastics were aimed at curbing sources of microplastics to the environment. In 2015, the Federal government passed the Microbead-Free Waters Act of 2015, which became Public Law 114-114. It prohibited “the manufacturing, packaging, and distribution of rinse-off cosmetics containing plastic microbeads” (U.S. Food and Drug Administration, 2023). In addition, eight States and multiple local governments have introduced motions to reduce sources of secondary microplastics by banning the use of single-use plastic bags in shops and grocery stores, and some cities have done the same for plastic straws (Usman and others, 2022).
Other legislation is aimed at monitoring and managing microplastics in drinking and ocean waters. California passed two bills in 2018 to establish standard definitions and methodology for monitoring microplastics and to address ecological risk from microplastics. California Senate Bill 1422 (SB–1422) required the Water Resources Control Board to “adopt a definition of microplastics in drinking water” and to “adopt a standard methodology to be used in the testing of drinking water” (State of California, 2017b). This standard methodology was subsequently be used for 4 years to test drinking water for microplastics, resulting in public disclosure of results. California Senate Bill 1263 (SB–1263) required a newly formed California Ocean Protection Council to “adopt and implement a statewide microplastics strategy,” (State of California, 2017a); the strategy was released in 2022 (California Ocean Protection Council, 2022). Minnesota Department of Health was directed to sample microplastics in surface and groundwater in the State (Minnesota Department of Health, 2019), and the USGS is leading the effort. Future legislation on microplastics at all levels of government is either pending or introduced (for example, Save our Seas 2.0 Act [33 U.S.C. 4201 note]), and continued focus on the issue may be expected in the coming years. There are no health risk levels or regulatory thresholds (for example, minimum risk levels, maximum contaminant levels) that have been set by the U.S. Environmental Protection Agency (EPA) or other regulating agencies for microplastics in drinking water (Sorensen and others, 2023).
USGS microplastic-related research is directed by public concerns and fundamental science questions. Resource managers and the public throughout the United States rely on the USGS to lead studies (for example, environmental monitoring) on local to national scales across multiple ecosystems (land, freshwater, and marine). Scientists at the USGS have broad expertise in both fate and characterization of chemicals and chemical mixtures, as well as environmental and ecosystem health; thus, it is one of the few Federal agencies equipped to engage in comprehensive scientific research on microplastics. Interest in determining the sources, pathways, fate, and biological effects of microplastics continues to grow among stakeholders with whom the USGS interacts. The USGS participates in an advisory capacity to local, regional, and Federal working and interest groups on microplastics and nanoplastics, such as the Chesapeake Bay Scientific and Technical Advisory Committee’s Plastic Pollution Action Team (Chesapeake Bay Program, 2024), the International Joint Commission’s Great Lakes Microplastics Working Group (Hataley and others, 2023), and the U.S. Government Nanoplastics Interest Group (National Nanotechnology Initiative, 2024), which provides insight based on the latest USGS research and coordinates the need for better science. While much is still unknown with respect to human and ecological implications associated with the various types, shapes, and chemical composition of microplastics in the environment, this science vision document serves to identify current (2023) gaps in microplastic knowledge and science needs and provide a framework for how USGS capabilities can address those gaps.
Recent, but limited, work by the USGS has focused on the occurrence of microplastics in water bodies, precipitation, and biota (Baldwin and others, 2016; Lenaker and others, 2019; Wetherbee and others, 2019; Baldwin and others, 2020; Baldwin and others, 2021; Lenaker and others, 2021). These efforts demonstrated the potential for bringing existing expertise and capabilities across the USGS to address microplastic science gaps. These efforts also demonstrated unique opportunities for the USGS to build on and expand our expertise, capabilities, and capacities to address a broader range of environmental microplastic science topics of national significance. As the USGS seeks to expand environmental and analytical capabilities for assessing microplastics in the environment, coordination with other Federal agencies involved in studying plastic pollution and the effects of plastics on human and ecological health will be critical. Open dialogue with the EPA Office of Research and Development, National Oceanic and Atmospheric Administration (NOAA) Marine Debris Program, National Institute of Standards and Technology (NIST), and U.S. Food and Drug Administration has been essential for sharing research and field methods and determining the USGS role in the Federal Government’s response to microplastic pollution.
2.0. Science Needs, Data Gaps, and Opportunities for Microplastic Research
2.1. Environmental Sources, Pathways, and Fate
Microplastics have been documented in every environmental compartment due to the ubiquitous use of plastics, their environmental persistence, the global connectedness of aquatic environments, and the long-range transport of microplastics. Microplastic sources and environmental pathways are diverse. While some of them may be easily identified and mitigated, identifying microplastic sources and pathways in an entire ecosystem or watershed is a significant challenge for researchers and resource managers. For instance, how do you prioritize and mitigate multiple inputs from land, water, and air? A better understanding of the relative contributions and characteristics of different microplastic sources and pathways and the factors affecting the fate of microplastic particles in the environment can inform policy and mitigation strategies (fig. 1).
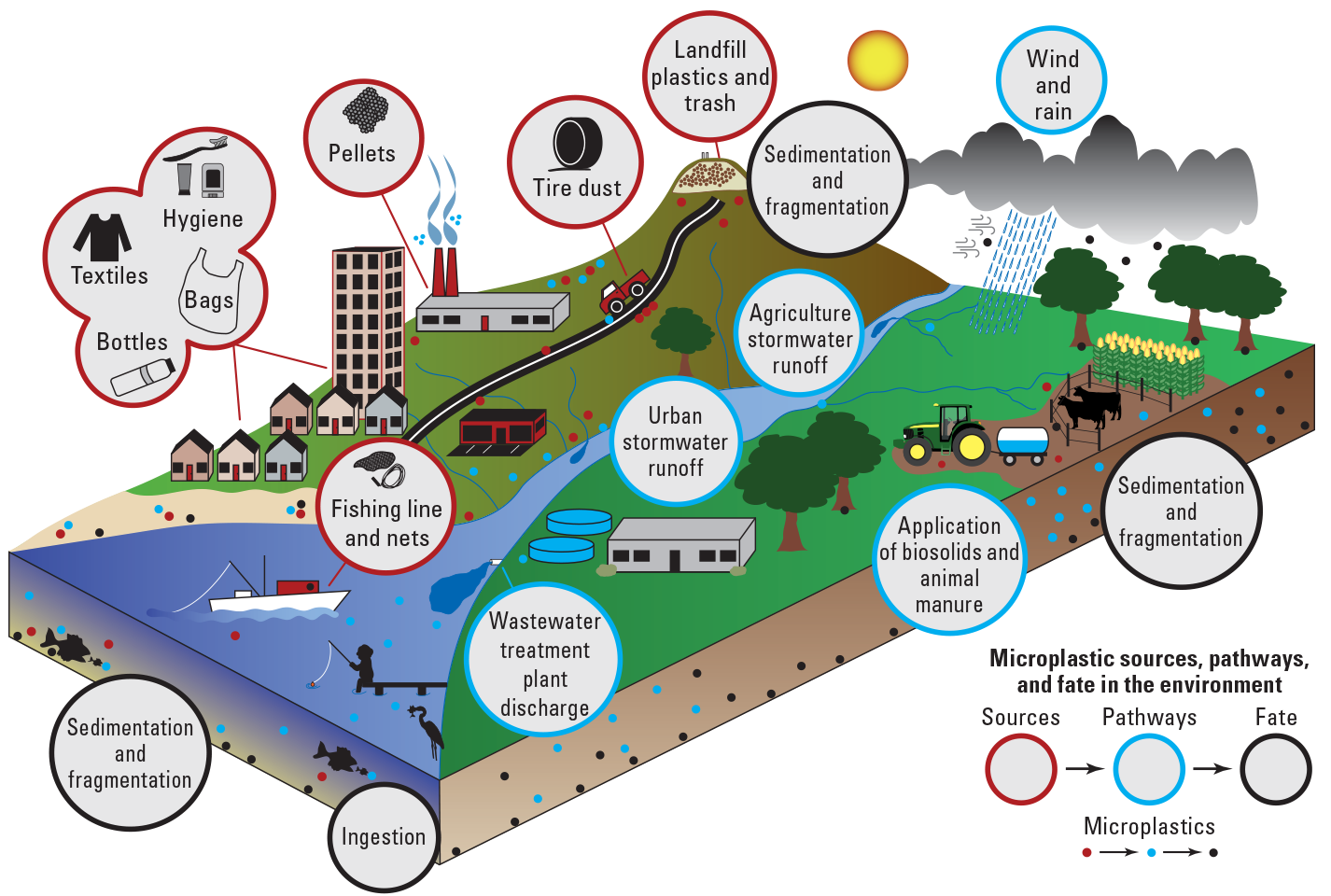
Conceptual diagram of microplastic sources, pathways, and fate in the environment.
Sidebar 4. Plastic Types That Are Common Sources of Microplastics And Example of Items Using Each Type
The list below gives the polymer names for types of plastics that are common sources of microplastics. Common uses of each type are modified from Driedger and others (2015).
-
• Acrylonitrile butadiene styrene: electrical cases, toys, drainage pipes, medical uses
-
• Polyamides/nylons: fibers, tubing, fishing line
-
• Polycarbonate: lenses include eyeglasses, helmets, headlights and windows
-
• Polyester: fibers and textiles
-
• Polyethylene, including high-density, low-density, and terephthalate: consumer goods, food and product packaging, medical use
-
• Polypropylene: food containers, car parts, pipes, bottle caps
-
• Polystyrene: foam products, packaging material, disposable tableware
-
• Polyurethanes: foams (cushioning and insulating), surface coatings, industrial usage
-
• Polyvinyl chloride: plumbing and gutters, window frames, flooring
-
• Polyvinylidene chloride: plastic wraps
-
• In addition, there are several plastic combinations (for example, polycarbonate plus (+) acrylonitrile butadiene styrene, polyethylene+acrylonitrile butadiene styrene), and proprietary and specialty formulations (for example, Teflon/PTFE, polymethyl methacrylate, epoxy, synthetic tire rubber).
2.1.1. State of the Science
2.1.1.1. Microplastic Sources
Primary microplastics are those that are manufactured in the microplastic size range and include scrubbers or microbeads used in personal care products, abrasive materials used in cleaning and air-blasting products, and feedstocks used in plastic manufacturing (resin powder or nurdle pellets, see sidebar 1). Secondary microplastics are fragments from the breakdown of larger plastics through physical, chemical, or biological processes. Sources of secondary microplastics include synthetic fibers shed from textiles (clothing, carpets, upholstery) during general use, washing, and drying (Hartmann and others, 2019; Kapp and Miller, 2020; Tao and others, 2022), and breakdown of larger plastics including fishing equipment, car tires (through road wear and reuse applications in artificial turf and rubberized pavements), agricultural plastics and mulches, and single-use items such as bags, bottles, cups, takeout containers, straws, cigarette butts, and medical waste, among many others (Hidalgo-Ruz and others, 2012; Eerkes-Medrano and others, 2015; Duis and Coors, 2016; Alimi and others, 2018; Zangmeister and others, 2022). Further breakdown of plastics from these and other sources yields nanoplastics. For example, research has documented that single-use consumer plastic products (such as single-use bags and beverage cups) release trillions of nanoplastic particles per liter into water during normal use (Zangmeister and others, 2022). Sidebar 4 lists plastic types that are common sources of microplastics and example of items using each type.
2.1.1.2. Microplastic Pathways
Microplastics reach aquatic and terrestrial environments through pathways such as wastewater, stormwater runoff and sewage overflow, and the atmosphere. Wastewater represents two important pathways: wastewater treatment facility effluent (that is, microplastics in treated liquid waste) and biosolids (that is, microplastics in treated solid waste) (Edo and others, 2022). Many studies have shown that wastewater treatment facilities remove almost all microplastics from influent water (95–99 percent removal; Carr and others, 2016; Talvitie and others, 2017), yet wastewater effluent still represents a significant pathway for microplastics to receiving waters because of the high concentrations of microplastics in the influent, and the large volume and continuous flow of effluent (Rochman and others, 2015; Mason and others, 2016; Talvitie and others, 2017). The wastewater biosolids, or sludge, captured by treatment facilities are an additional and distinct pathway for microplastics to the environment (Edo and others, 2022). Biosolids contain thousands to tens of thousands of microplastic particles per kilogram (kg) dry weight (Mahon and others, 2017), and roughly 50 percent of the 730 million kg of biosolids collected annually in the United States are land-applied on agricultural fields, forests, reclamation sites, and lawns and gardens (U.S. Environmental Protection Agency, 2016). Microplastics in land-applied biosolids may be lofted to the air by wind, incorporated into soils, or enter waterbodies through runoff. Estimates of the microplastic burden on soils in North America and Europe due to biosolids application exceed estimates of the burden in surface waters of the global oceans (Nizzetto and others, 2016). Research from Australia estimates that 4,700 metric tons (t) of microplastics are released into the Australian environment annually through land application of biosolids (Okoffo and others, 2020). In the United States, irrigation diversions from streams represent an additional pathway for microplastics to the terrestrial environment (Kukkola and others, 2023).
Stormwater runoff and sewage overflow systems represent another important pathway for microplastics to waterways (Werbowski and others, 2021; Zhu and others, 2021). Concentrations of microplastics in stormwater exceed those in wastewater effluent by multiple orders of magnitude (Sutton and others, 2019; Werbowski and others, 2021; Zhu and others, 2021). Watershed-scale comparisons of microplastic loading from different pathways are limited, but a study of microplastic loading to San Francisco Bay estimated stormwater loads to be 300 times greater than those in wastewater effluent (Sutton and others, 2019). These estimates are almost certainly affected by local land cover, terrain, stormwater treatment practices, and climate characteristics, and thus, may not reflect relative load contributions in other locations but serve to highlight the importance of stormwater as a pathway for microplastics to reach aquatic environments. Preliminary studies indicate that bioretention cells, rain gardens, and other stormwater retention structures can be effective tools for reducing microplastic loads in stormwater, capturing 83–96 percent of particles (Gilbreath and others, 2019; Smyth and others, 2021; Werbowski and others, 2021).
The atmosphere is an additional pathway through which microplastics reach aquatic and terrestrial environments. Microplastic fibers and fragments are lofted into the atmosphere from roads, the ocean (through wind and wave action), agricultural fields, and population centers (Brahney and others, 2021), and once aloft, may be transported long distances (Allen and others, 2019; Bergmann and others, 2019). Atmospheric deposition of microplastics occurs in all landscapes, even remote areas such as the Alps (south-central Europe), the Arctic (above 66.5 degrees north latitude), and protected areas of the western United States (including hard-to-reach areas in Yellowstone, Alaska, and the Rocky Mountain region), at rates ranging from 50 to 700 particles per square meter per day (m2/d) (Cai and others, 2017; Dris and others, 2017; Allen and others, 2019; Bergmann and others, 2019; Wetherbee and others, 2019; Brahney and others, 2020a). An estimated 22,000 t of microplastics are deposited on the contiguous United States each year (Brahney and others, 2021).
2.1.1.3. Microplastic Fate
Microplastic transport in aquatic systems is affected by many factors, including buoyancy (specific gravity), size, and shape, which determine whether a microplastic particle will settle or remain in the water column (Horton and Dixon, 2018). Environmental considerations such as flow velocity, turbulence, wind, currents, and wave action also affect microplastic movement and transport (Horton and Dixon, 2018). The chemical characteristics of the water further affect whether a microplastic particle or fiber will float on the surface, suspend in the water column, or deposit in bed sediment. The formation of biofilms on microplastic surfaces, aggregation with other microplastics and sediment particles, and interaction with organic matter also play a role in transport properties, deposition, and potential for resuspension (Semcesen and Wells, 2021).
Weathering processes (photodegradation, thermal degradation, biodegradation, and mechanical fragmentation) can change the physical shape, size, and chemical properties of microplastics, ultimately affecting microplastic fate (Duan and others, 2021). Some microplastics may be slowly biodegraded by micro-organisms (Eerkes-Medrano and others, 2015; Rocha-Santos and Duarte, 2015), although even degradable plastics remain in the environment for decades to centuries (Barnes and others, 2009; Roy and others, 2011). Some plastics are more susceptible to oxidative weathering by ultraviolet light (photodegradation), although rates vary based on water column or soil mobility considerations that affect intensity (light penetration at depth; Hebner and Maurer-Jones, 2020; Masry and others, 2021). Microplastics can be mistaken for edible material by wildlife, or simply swallowed with surrounding water. While some microplastics may become trapped in the guts and gills of organisms, others may fragment or undergo surface degradation by digestive enzymes (Dawson and others, 2018). These different weathering processes affect microplastic surface properties (creating cracks, for example), which can lead to increased adsorption of heavy metals and organic contaminants, and fragmentation into smaller particles (Duan and others, 2021; Bhagat and others, 2022; Hadiuzzaman and others, 2022). With fragmentation, microplastics become more prevalent, surface area increases relative to volume, and particles become more bioavailable to organisms at the base of the food web (Botterell and others, 2019).
In aqueous environments, microplastics float or settle depending on particle density, size, shape, degree of surface biofouling, and waterbody hydraulics (Cowger and others, 2021; Semcesen and Wells, 2021). However, even low-density microplastics will eventually sink with increasing fragmentation and surface biofouling (Peng and others, 2020; Semcesen and Wells, 2021). For this reason, and based on microplastic concentrations in these environments, streambeds, lakebeds, and sea floors are often considered the ultimate sinks for microplastics (Woodall and others, 2014; Peng and others, 2020; Lenaker and others, 2021; Drummond and others, 2022).
In terrestrial environments, soils are a sink for microplastics accumulated through atmospheric deposition, land application of reuse materials (for example, biosolids, livestock manure), and mismanagement and breakdown of trash (He and others, 2018; Brahney and others, 2020a; Jin and others, 2022; Tagg and others, 2022). However, soils may only be a temporary sink, as wind may resuspend microplastics into the atmosphere, runoff may wash microplastics into a nearby waterway, or microplastics may infiltrate into groundwater (Grbić and others, 2020; Brahney and others, 2021; Bigalke and others, 2022; Rezaei and others, 2022).
Throughout the microplastic cycle, microplastics can act as both a source and a sink for chemical pollutants. Compounds added to plastics during the manufacturing process (for example, flame retardants, plasticizers, antibacterial agents, ultraviolet inhibitors, reaction byproducts) can leach out of plastics (Schrank and others, 2019; McIntyre and others, 2021). Conversely, microplastics may absorb pollutants in aquatic and terrestrial ecosystems, potentially concentrating persistent, bioaccumulative and (or) toxic organic and microbiologic pollutants and metals (Imran and others, 2019; Wang and others, 2021a; Hu and others, 2022). The absorption and desorption of chemical contaminants from microplastics may create regional hotspots of chemical pollutants due to microplastic transport/deposition (Eerkes-Medrano and others, 2015) and (or) contribute to the bioaccumulation of hazardous compounds in aquatic and terrestrial organisms (for example, polybrominated diphenyl ether added to plastics as flame retardants; Rochman and others, 2014).
The forensics of source apportionment of microplastics is complicated by the pervasive and overlapping use of plastics for different applications. For example, polypropylene is used in packaging, construction, furniture, transportation, electronics, textiles, and many other application sectors (Di and others, 2021). Hence, identifying the source of a polypropylene particle found in the environment is a challenge. Microplastic morphology, additives, and surface contaminants including metals, organic contaminants, and biofilms may help identify microplastic sources and (or) pathways (Fahrenfeld and others, 2019). Microplastic assemblages may also provide clues: studies have found that waters from different pathways (stormwater runoff, agricultural runoff, and wastewater effluent) may have distinct signatures (Grbić and others, 2020; Zhu and others, 2021). Stormwater, for example, had a high proportion of black rubbery particles, presumably from car tires, whereas wastewater was dominated by fibers, a large proportion of which were cellulose, presumably from washing of clothes (Grbić and others, 2020; Zhu and others, 2021).
2.1.2. Science Gaps
Recent understanding of microplastic sources, pathways, and fate has improved considerably over the past decade, but many gaps remain. For example, a better understanding of the characteristics and relative importance of different microplastic sources and pathways in terrestrial and aquatic environments is needed to enable source apportionment and to inform management solutions. Few studies have examined microplastic characteristics (morphologies, sizes, polymers, additives, relative concentrations) from different sources and pathways on a watershed scale (Zhu and others, 2021). Holistic, multimatrix watershed- or basin-scale studies are needed to understand the characteristics and relative importance of microplastics from different sources and pathways. Municipal wastewater treatment facilities are known pathways for microplastics to the environment, but less is known about industrial wastewater contributions, including those from food, beverage, and feedstock processing facilities. Similarly, little is known about chemicals or chemical mixtures that co-occur with microplastics, or physical characteristics such as optical properties, that could be used as indicators of specific microplastic sources or pathways.
In terrestrial environments, biosolids and atmospheric deposition are known contributors of microplastics, but their relative importance and defining characteristics of these pathways are not well understood, and even less is known about other microplastic sources and pathways to terrestrial environments, such as agricultural wastes and drilling fluids. The fate of terrestrial microplastics is also unclear. Neither weathering of microplastics in terrestrial environments, nor the flux of terrestrial microplastics to surface waters, groundwater, or the atmosphere are known.
Finally, the scientific community needs a better understanding of microplastic mitigation strategies. In a few studies, researchers have examined microplastic load reduction using stormwater control measures (green infrastructure) such as bioretention cells and rain gardens and have demonstrated promising results (Gilbreath and others, 2019; Smyth and others, 2021; Werbowski and others, 2021). However, these studies were fairly small, local, and limited in the types of green infrastructures tested. A more comprehensive assessment of mitigation strategies would help fill this important gap.
2.1.3. USGS Capabilities and Expertise
Expertise within the USGS can help fill many of the science gaps related to microplastic sources, pathways, and fate. The USGS has extensive expertise in sampling and modeling a wide variety of point and nonpoint source contaminants, including microplastics, in stormwater, wastewater, agricultural runoff, atmospheric deposition, and other pathways. The USGS also manages large monitoring networks of streamgages, groundwater wells, atmospheric deposition sites, and ecological sites across the country. To date, the utilization of USGS expertise and monitoring networks for microplastic research has been limited to a relatively few local and regional studies. By applying its expertise more deliberately, and through greater leveraging of its nationwide monitoring networks, the USGS can answer holistic, watershed- to national-scale questions about the characteristics and relative importance of microplastics from different sources and pathways. Active partnerships with other agencies and their monitoring networks can be used to expand this research. Characterizing changes in microplastic particle characteristics (dimensions, density, sorption/leaching of chemicals, biofouling, surficial cracking) during weathering and transport, which can affect microplastic toxicity (Duis and Coors, 2016), is also a capability of the USGS and academic partners.
2.1.4. USGS Science Opportunities
2.1.4.1. Short-Term Opportunities
Most of the science opportunities related to microplastic sources, pathways, and fate require USGS access to a microplastics laboratory, either internal or external, with the capability of providing microplastic quantification and polymer identification for complex environmental matrices; for example, stormwater, biosolids, sediment, soil, and biological tissue (table 1). The laboratory needs to handle large numbers of samples and meet USGS quality-assurance standards. The USGS has the expertise and experience to develop these capabilities. Thus, in the short term (1–2 years), the USGS could begin to address several of the microplastic science gaps identified in this section by designing studies aimed at addressing science gaps, beginning sample collections, purchasing laboratory equipment, and developing analytical capabilities, but study completion would likely be a longer term effort requiring more than 2 years.
2.1.4.2. Long-Term Opportunities
There are several long-term opportunities for the USGS to help fill science gaps related to microplastic sources, pathways, and fate (table 1). For example, the USGS could leverage its expertise and national monitoring networks for holistic, multimatrix watershed-scale evaluations of microplastic sources and pathways in different regions to determine the relative importance of different sources and pathways and inform source and pathway apportionment. Additionally, the USGS could assess microplastic mitigation and sequestration strategies across a variety of natural and built environments (for example, green infrastructures); evaluate natural and anthropogenic drivers that deliver microplastics to groundwater systems; investigate chemical sorption and desorption of contaminants in environmental matrices and leaching of chemicals to determine the relative effect compared to contaminants in other environmental compartments; characterize the fragmentation/breakdown properties of different polymers into smaller size fractions under varied environmental conditions; and determine how the fate of microplastics and microplastic-associated contaminants is affected by natural organic matter, micro-organisms, and growth of biofilms on microplastic surfaces.
Table 1.
Short- and long-term science opportunities for researching environmental sources, pathways, and fates of microplastics.[Short- and long-term science opportunities determined through literature reviews and gap analyses. USGS, U.S. Geological Survey]
2.2. Human and Wildlife Exposure Routes
Exposure routes for microplastics in humans and wildlife include ingestion (active or passive, through contaminated food or water, fluvial particles, and deposited dust), inhalation (lungs, gill), and dermal contact (Prata and others, 2020; Roch and others, 2020). Microscopic assimilation, ingestion, and presence in the gut or tissue of many organisms in laboratory and field studies have been documented across all trophic levels, including phytoplankton, zooplankton, algae, plants, invertebrates, fish, amphibians, reptiles, birds, and mammals. Once in the food web, microplastics can transfer to higher trophic levels. Humans are primarily exposed to microplastics through inhalation and ingestion (Prata and others, 2020), and microplastics have been found in lung tissues (Jenner and others, 2022) and in blood (Leslie and others, 2022). Although the USGS focuses on the effects of microplastics on the environment and wildlife, we also work closely with State and Federal agencies and other partners that focus on human health to develop integrated holistic One Health Science.
2.2.1. State of the Science
2.2.1.1. Environmental Exposure
Early research on the presence of microplastics in the environment was completed in aquatic ecosystems, with marine studies (77 percent) dominating (de Sá and others, 2018; Miller and others, 2020). In recent years, studies have expanded greatly into freshwater and terrestrial ecosystems (Eerkes-Medrano and others, 2015; Sarijan and others, 2021; Wong and others, 2020). Ingestion of food and water are considered the most important pathways of microplastic uptake for aquatic and terrestrial wildlife and humans (Prata and others, 2020; Roch and others, 2020; Zantis and others, 2021). However, a recent study reviewing ingestion of table salt, drinking water, and inhalation of air as exposure routes indicated inhalation (indoor air) to be the most significant pathway for human exposure (Zhang and others, 2020). In addition, fish (Kiryu and others, 2000; Lu and others, 2016; Bhagat and others, 2020) and aquatic invertebrates (Watts and others, 2014; Kolandhasamy and others, 2018) can also absorb microplastics through the skin, gills, and other exposed tissue. Microplastics in aqueous exposures attached to the chorion of zebrafish (Danio rerio) eggs but were not observed in the embryos (Batel and others, 2018). To date, no clear evidence of maternal transfer has been demonstrated in higher vertebrates, although microplastics were observed in human placentas (Ragusa and others, 2021).
Accidental ingestion may be common for passive filter feeders and for active foragers such as fish and some invertebrates, which may mistake the particles for food (Roch and others, 2020). In a laboratory study, fish species relying on visual foraging cues ingested more microplastics than those relying on chemosensory cues (Roch and others, 2020), which may explain why many pelagic fish species have been reported with higher concentrations than benthic species in the marine environment. However, in the riverine environment, benthivores may have an even higher risk than plankivores due to accumulation of microplastics in sediments (McNeish and others, 2018). Diet breadth may also affect microplastic uptake. In a study of intertidal fishes, omnivorous species had higher amounts of microplastics in their guts than herbivores or carnivores (Mizraji and others, 2017).
2.2.1.2. Translocation Within Organisms
The potential for bioaccumulation (accumulation within the tissues of an organism at a level greater than the surrounding environment) of microplastics seems to be variable according to current (2023) literature. Gouin (2020) reviewed data from more than 800 organisms at all levels of biological organization in which microplastics have been observed. Approximately 90 percent of the studies enumerated microplastics in the stomach and (or) gastrointestinal tract. It is documented that the presence of microplastics in the gut is often transient as both ingestion and egestion or excretion of microplastics occurs (Woods and others, 2018; Xiong and others, 2019). Assessment of the data did not support bioaccumulation or biomagnification. Conversely, McIlwraith and others (2021) found that seven species of sportfish showed widespread presence of microplastics in the guts, livers, and fillets. Although microplastics in the gut may be excreted, in 2023, it is unknown if particles in other tissues will be as easily removed. In a review and meta-analysis of studies in the marine environment, Miller and others (2020) indicated that bioaccumulation occurs within trophic levels, but biomagnification (increasing concentrations with increasing trophic level) was not observed. The bioaccumulation appeared to be more strongly related to feeding strategies than trophic levels. More information is needed about how microplastics enter specific tissues and if they remain there.
2.2.1.3. Translocation of Microplastics Among Organisms
Trophic transfer (the transfer of contaminants from one trophic level to another) of microplastics in simple food chains has been documented. For instance, consumption of contaminated zooplankton resulted in the translocation of microplastics to benthic filter feeders (Van Colen and others, 2020). Predatory midges were also found to accumulate microplastics through consumption of larval (Culex) mosquito prey (Cuthbert and others, 2019). Microplastics can also become entrapped in biofilms and then become available to biofilm grazers (McCormick and others, 2016). Microplastics adhered to or within biofilms on aquatic plants can be a route of exposure when the plants are eaten by herbivorous invertebrates and fishes (Gutow and others, 2016; Goss and others, 2018). Zhang and others (2019) measured microplastic abundance in 11 wild fish species and 8 wild crustacean prey species and found that abundance was significantly lower in crustaceans than fish species, leading to speculation that trophic transfer occurred.
The mechanisms driving microplastic trophic transfer in more complex food webs is relatively unknown (Provencher and others, 2019; Krause and others, 2021). A study evaluated microplastic transfer from water to tadpoles to fish to mice. Tadpoles ingested and accumulated microplastics from the water, and fish that ate those tadpoles had microplastics not only in the gut but also the liver. Microplastics were also observed in the livers of mice fed ground contaminated fish (da Costa Araújo and Malafaia, 2021).
Shifts in habitat caused by ontogenetic changes in animals (that is, changes in appearance, habitat, or diet, during its development) could also be important in mediating movements of microplastics within and across ecosystem boundaries. Foraging movements, migrations, and other ontogenetic shifts in habitat such as caused by insect metamorphosis can alter distribution and exposure to microplastics. Fish and bird migrations can move particles long distances from the source and between aquatic and terrestrial ecosystems (Bourdages and others, 2021). Microplastic beads in larval aquatic insects, such as mosquitos, can be transferred from larvae to pupae to adult, although the number of particles in adults are greatly reduced (10–100 times lower) compared to numbers in pupae or larvae (Al-Jaibachi and others, 2018). Because Culex oviposition, habitat selection, growth, and survival through metamorphosis were not affected by microplastic accumulation, even a small per individual transfer of microplastics could export a large quantity of microplastics from aquatic ecosystems to the terrestrial environment (Al-Jaibachi and others, 2019). Microplastics adhered to the periphyton can be ingested by tadpoles which, through metamorphosis, could be another transfer path from the aquatic to terrestrial environment (Boyero and others, 2020). Finally, feces and decomposition of plant and animal tissue can be another pathway of microplastic movement within and among ecosystems. For instance, filter-feeding benthic invertebrates can ingest microplastics from the water column and transfer them to benthic organisms through feces or pseudo feces (Krause and others, 2021).
2.2.2. Science Gaps
To understand exposure routes and sublethal effects (see section 2.3., “Ecotoxicology”) of microplastics, research is needed to identify the factors affecting uptake of microplastics and potential accumulation within various tissues and ecosystem components. The need for research is especially true in identifying routes of exposure for humans and other higher vertebrates. For instance, humans may consume multiple tissue types of certain organisms (for example, shellfish) but primarily the muscle of other organisms (for example, fish). Very little is understood about the mechanisms driving trophic transfer of microplastics and their movement through complex ecosystems. Additional information is needed on transfer among plants, algae, and other lower trophic levels, from invertebrates to vertebrates. There is also a need to understand transport among segments of an ecosystem, including air, water, and land. For example, the insect-mediated transfer of microplastics from aquatic to terrestrial environments could lead to additional unexpected exposures of terrestrial consumers to aquatically derived plastics.
2.2.3. USGS Capabilities and Expertise
The USGS has a broad history of research on the various routes of chemical exposure to wildlife and is well positioned to address the knowledge gaps surrounding exposure to microplastics. One area of strength is the cross-disciplinary work that has led to the analysis of many chemical compounds within tissues of both aquatic and terrestrial organisms collected as part of landscape or ecosystem studies. The infrastructure for analyzing these compounds in multiple sample types has been well demonstrated within the USGS, from multiple disciplines and within multiple mission areas (for example, ecosystems, water resources, and energy and minerals). Microplastic analyses could be added to samples from various media, including water, sediment, and tissue.
Predictive models of contaminant transfer have been developed for many situations: (1) transfers between aquatic and terrestrial environments and across insect metamorphosis (Kraus and others, 2014; Kraus, 2019; Krause and others, 2021) and (2) accumulations in sentinel taxa, including birds (Custer and others, 2003) and spiders (Walters and others, 2018). In addition, the USGS works closely with public health officials across the Nation to track data on human health.
2.2.4. USGS Science Opportunities
2.2.4.1. Short-Term Opportunities
The USGS is working with Federal agencies, State agencies, Tribes, nongovernmental organizations, and academic institutions to expand its research on human and wildlife exposure routes from microplastics (table 2). One short-term opportunity would be the derivation of a predictive framework for understanding the routes of microplastic exposure to wildlife based on previous knowledge of environmental transport and bioaccumulation of contaminants known to adsorb to particles. In addition, the USGS and its partners could study trophic and ontogenetic transfer, which would include the transfer of microplastics and degraded compounds among organisms, as well as determining the effects of migratory and ontogenetic movements on the transport of microplastics from place to place.
2.2.4.2. Long-Term Opportunities
The USGS could contribute to and (or) start collaborative interagency or multi-institution research studies that would identify exposure routes of microplastics that have not been adequately researched. Paired laboratory and field studies of the patterns of uptake and transfer of microplastics and their associated chemicals would reveal causal mechanisms and landscape patterns of exposure to microplastics (table 2).
Table 2.
Short- and long-term science opportunities for researching potential exposure routes of microplastics to humans and wildlife.[Short- and long-term science opportunities determined through literature reviews and gap analyses. USGS, U.S. Geological Survey]
2.3. Ecotoxicology
Microplastics have been documented in many species worldwide, yet ecotoxicological data for microplastic exposures are relatively sparse (Anbumani and Kakkar, 2018; Prokić and others, 2019; Huang and others, 2020). However, the effects of microplastics have been documented in organisms from bacteria to mammals and toxicology studies show that these effects are complicated. Toxic effects of microplastics, when found, have been attributed to (1) the particles themselves (Wright and others, 2013; Anbumani and Kakkar, 2018), (2) intrinsic chemicals associated with plastics during manufacturing, and (3) exogenous compounds for which the microplastics are vectors of transport into the organism. Effects have been studied most commonly at the organism or suborganismal level, but ultimately it is important to understand effects at the population, community, and ecosystem levels.
2.3.1. State of the Science
Recent reviews of the state of the science regarding the ecotoxicological effects of microplastics (Eerkes-Medrano and others, 2015; Anbumani and Kakkar, 2018; de Sá and others, 2018; Prokić and others, 2019; Huang and others, 2020; Ma and others, 2020; Sarijan and others, 2021) report effects on survival, growth, reproduction, immune response, metabolic changes, and behavior. Among reports of microplastics in aquatic environments, most have been on organisms such as fish and small invertebrates (de Sá and others, 2018). Meta-analysis of the research on those species indicate that species have neutral to slightly negative effects of microplastics on consumption, growth, reproduction, and survival that vary among taxa (Foley and others, 2018). Toxicity data for other species, including amphibians, mammals, reptiles, and birds, are limited and require further study. A short review of effects of microplastics on sea birds speculates that microplastics may negatively affect feeding and nutrient absorption on sea birds such as the Antarctic prion (Pachyptila desolata), sooty shearwater (Puffinus griseus), giant petrel (Macronectus giganteus) according to Susanti and others (2020). In humans, microplastics have been implicated in the disruption of immune function and neurotoxicity. The immune system’s inability to remove the particles may lead to oxidative stress, chronic inflammation, and increased risk of neoplasia (Prata and others, 2020).
2.3.1.1. Toxicity of Microplastic Particles
The toxicity of microplastics is concentration dependent (Gutow and others, 2016; Lu and others, 2016; Everaert and others, 2018) and varies based on the type, size, and shape of the particles (Huang and others, 2020; Zimmermann and others, 2020). Generally, smaller particles are more toxic than larger ones because they have greater surface-area-to-volume ratios and can translocate through cellular membranes (Jeong and others, 2017). However, this pattern is highly reliant on the mechanism of effect (for example, false satiation leading to reduction of food ingestion) versus physiological response (damage to gut walls and hypoxia), species morphology, and life stage (Martins and Guilhermino, 2018; Wu and others, 2019).
Microplastics are toxic to primary producers such as phytoplankton, disrupting feeding and photosynthesis, and negatively affecting growth, development, and reproduction. Additionally, polysaccharides secreted by phytoplankton may agglomerate (form into a mass) microplastics (Long and others, 2017), creating an exposure pathway for higher trophic species. Copepods (Calanus helgolandicus) feeding in the presence of microplastics consumed 40 percent less carbon biomass, resulting in lower growth, fecundity, and survival (Cole and others, 2015). Corals may be affected both through ingestion and passive adherence, leading to adverse effects on coral cleaning and feeding mechanisms. Soares and others (2020) documented many effects, including reduced growth, decreased activity of detoxifying and immunity enzymes, and negative effects on coral-Symbiodiniaceae relationships.
Exposure of insects such as honeybees (Apis spp.) to microplastics in food affected their gut microbiome, leading to decreased gut microbiota α-diversity (Wang and others, 2021b). These changes altered the expression of certain genes, including some related to the immune system, although bee growth was not affected. Dosing the bees with antibiotics greatly increased the lethality of the microplastics to the bees, leading the authors to indicate that the bee gut microbiome protects the bees from the toxic effects of microplastics (Wang and others, 2021b). Alterations of gut microbiomes of other invertebrates such as shrimp (Duan and others, 2021) have also been reported. Studies have also documented aquatic vertebrates, such as zebrafish, with intestinal inflammation and disorders of the metabolome (Qiao and others, 2019; Roch and others, 2020).
Microplastics in organisms such as fish and aquatic invertebrates affect feeding, growth, development, immune response, reproduction, and survival, and they can cause tissue inflammation (Foley and others, 2018; Ahrendt and others, 2020; Horn and others, 2020). The presence of microplastics is often evaluated in the stomach and gastrointestinal tracts, and correlations are made between presence of microplastic particles and observed effects. While it has been documented that microplastic particles can be absorbed from gut, gill, and body surfaces and transported to other tissues, many species quickly eliminate certain types and sizes of microplastics (Graham and others, 2019; Spanjer and others, 2020). The uptake of particles from the gut and translocation are related to particle size. Laboratory studies with zebrafish indicated that microplastic particles (<5 μm) were translocated to the liver (Lu and others, 2016). More toxicity data are needed at environmentally relevant concentrations and for environmentally relevant particle types.
2.3.1.2. Toxicity of Intrinsic Chemicals
During manufacturing, chemical additives such as metals and organic chemicals are often added to plastics to improve performance, elasticity, slow degradation, or reduce flammability, among other purposes. Chemical additives include, but are not limited to, flame retardants, phthalates, phenols, bisphenol A, antimicrobials, and synthetic antioxidants. Some of these additives may be endocrine disrupting and (or) toxic to organisms if they later leach from plastics into the environment or directly into their digestive tracts (Chen and others, 2022). Understanding the role of additives on the environment and wildlife is critical.
Multiple studies on aquatic organisms, including invertebrates, fish, and waterbirds, have shown the synergistic negative effects of microplastics and adsorbed contaminants, including changes in blood chemistry, foraging and swimming behavior, genotoxicity, oxidative damage to cells, metabolic activity, immunotoxicity, neurological responses, alterations in gene expression, liver toxicity, and pathology after chronic exposure (Huang and others, 2021). Leaching of these metals and organic compounds into the environment depends on the physicochemical properties of the chemicals and can change due to a range of factors, including salinity, hydrophobicity and (or) lipophilicity, molecular weight, surface charge, water or air velocity, and exposure to sunlight (Chen and others, 2019a). For example, ultraviolet radiation accelerates the release of polybrominated diphenyl ethers (Khaled and others, 2018), while salinity slows the leaching behavior of phthalates (Paluselli and others, 2019). Microplastics collected from the marine environment continued to leach endocrine-disrupting chemicals (for example, multiple phenols) when exposed to solar irradiation for 1 month (Chen and others, 2019b). Other researchers have demonstrated the leaching of toxic chemicals from tire rubber particles, including 6PPD-quinone, polyaromatic hydrocarbon congeners, benzothiazole, and zinc (Panko and others, 2013; Redondo-Hasselerharm and others, 2018; Capolupo and others, 2020; Kolomijeca and others, 2020; Tian and others, 2021).
Ingested plastics can leach chemicals directly into the gastrointestinal tract of consumers through the desorption of intrinsic contaminants. Estrogenic and polybrominated diphenyl ethers chemicals were shown to leach from multiple plastic types, including polypropylene, polystyrene, and polyethylene (see sidebar 4), when placed in laboratory mimics of gastrointestinal conditions of both fish and sea birds (Coffin and others, 2019; Guo and others, 2020), but co-ingestion of sediment and food items can confound the amount of leachate that makes it into the organism through the adsorption of these leached chemicals. Additional work has shown that multiple metals leach from ingested plastics, including bromine, cadmium, chromium, mercury, lead, and antimony, and that their mobilization in the gut fits standard diffusion models (Smith and Turner, 2020). Although many chemicals leach into the gut after ingestion, little work has demonstrated acute or chronic toxicity or determined if microplastics represent a pathway for intrinsic plastic chemical additives that bioaccumulate and biomagnify at environmentally relevant microplastic concentrations.
The exposure to and effects of leached microplastic chemicals on organisms can vary. Microplastics can act as both a vector (delivering contaminants to an organism) and a scavenger (restricting the uptake of contaminants to an organism) of organic contaminants, dependent on the concentration of both the additives and the plastic (Liu and others, 2020). Field studies have correlated microplastic uptake with chemical concentrations of leachate in tissues (Jang and others, 2016; Khoshmanesh and others, 2023). Laboratory studies have documented the potentially acute toxic effects of leached plastic additives (both organic and metal) on aquatic organisms through leaching experiments (Huang and others, 2020). However, the presence of microplastics in the gut along with exposure to different chemical additives can have confounding effects, with plastics acting both to promote (Wardrop and others, 2016) and prevent (Chua and others, 2014) the uptake of organic contaminants.
2.3.1.3. Vector for Exogenous Compounds
In addition to acting as a vector of leached plastic additives, microplastics are shown to effectively concentrate contaminants, including organic contaminants and metals, from their surrounding medium (that is, air or water) (Brennecke and others, 2016; Liu and others, 2019). The concern about microplastics acting as a vector for exogenous compounds is twofold: microplastics can act as vectors for these adsorbed contaminants and absorbed substances may increase microplastics uptake. For example, Athey and others (2020) found that larval fish more readily consumed zooplankton prey containing microplastics that had been treated with DDT (dichloro-diphenyl-trichloroethane) than they consumed zooplankton that contained untreated plastics.
Factors affecting the toxicity of adsorbed contaminants are similar to factors affecting contaminants that leach out from virgin (that is, newly manufactured) plastics. There are potentially thousands of contaminants that can be adsorbed to microplastic particles. The type of contaminants adsorbed by microplastics is dictated mainly by a chemical’s hydrophobicity (though, pi interaction and electrostatic interaction are also important); more hydrophobic chemicals are more likely to adsorb to microplastic polymers (Tourinho and others, 2019; Luo and others, 2022). Multiple studies, on invertebrates, fish, and waterbirds, have shown the synergistic negative effects of adsorbed contaminants and microplastics, including changes in blood chemistry, changes in foraging and swimming behavior, genotoxicity, oxidative damage to cells, metabolic activity, immunotoxicity, neurological responses, alterations in gene expression, liver toxicity, and pathology after chronic exposure (Huang and others, 2020). Microplastics can also act as antagonists by adsorbing aqueous phase contaminants, which reduces organism exposure, even when those contaminant-laden plastics are ingested (that is, desorption in the organism does not occur) (Rehse and others, 2018). Results are highly chemical, plastic, and species specific, making it difficult to generalize the effect of exogenously absorbed contaminants on aquatic organisms. A critical review and re-interpretation of empirical studies by Koelmans and others (2016) indicates that in most marine habitats, consumers assimilated hydrophobic organic chemicals from natural prey tissues in much greater amounts than they assimilated from ingested microplastics; thus, microplastic ingestion is not likely to increase exposure to those chemicals.
2.3.1.4. Populations and Community Effects
By providing a novel substrate and potential source of energy and toxicants to micro-organisms, microplastics can change the assemblages and functions of microbe communities in the environment and guts of living organisms (Lu and others, 2019; Helmberger and others, 2020; Ma and others, 2020). These changes have implications for human and environmental health. For example, microbial colonization of microplastics in wastewater can possibly lead to the spread of antimicrobial resistance genes in biofilms in wastewater treatment plants (Eckert and others, 2018; Lu and others, 2019). Bacteria, fungi, and other micro-organisms can colonize microplastics, forming biofilms called the plastisphere (Zettler and others, 2013). A study indicated that plastics were home to taxonomically enriched and distinct fungal communities and accumulated pathogenic fungi within a riverine system (Xue and others, 2021). Prata and others (2019b) reviewed the effects of microplastics on microalgal populations and concluded that current (2023) environmental concentrations would not cause toxicity. However, disruption of populations could occur by reducing the availability and (or) absorption of nutrients or an increase in populations by decreasing predator populations such as zooplankton that may be more susceptible to microplastic toxicity (Foley and others, 2018).
Population effects of microplastics have also been documented in invertebrate species. Mueller and others (2020) documented changes in nematode population density in sediments exposed to microplastic beads. Martins and Guilhermino (2018) documented that exposure of Daphnia (small planktonic crustaceans) to ecologically relevant microplastic concentrations (polluted sites) led to mortality even beyond the exposed first generation. After a single exposure, succeeding generations of Daphnia exhibited lower fecundity and did not recover to preexposure condition until the third generation (Martins and Guilhermino, 2018).
Finally, predator-prey interactions can be affected by the presence of microplastics and their leachates, although the mechanisms and magnitude of effects (if any) are variable. A meta-analysis of reported effects of microplastics on fish and aquatic invertebrates found that a reduction in prey consumption was the most consistent effect detected across studies (Foley and others, 2018). In an experimental trial, predation rates of zooplankton larvae by benthic filter feeders (bivalve mollusks) were 30 percent lower when zooplankton were contaminated with microplastics than when clean prey were available (Van Colen and others, 2020). The microplastic uptake by zooplankton altered their swimming behavior and made them less likely to be filtered from the water column and consumed (Van Colen and others, 2020). In another experiment, microplastic leachates from virgin and bleached microplastic pellets impaired the vigilance and predator-avoidance behaviors of an intertidal snail (Littorina littorea) by approximately 15–64 percent when exposed to chemical cues of a common predatory crab (Carcinus maenas) (Seuront, 2018). These findings imply that consumption rates of these larval fish could potentially increase in the presence of microplastics. Consumption of mosquitoes by Chaoborus (midge) larvae (attack rate and handling time) was not affected by uptake of microplastic particles by mosquito prey (Cuthbert and others, 2019). Thus, different studies indicated that microplastics could make prey better or worse at avoiding predation or evoke no change. In terms of how these effects could play out at the population level, a modeling study extrapolated that reductions in feeding due to presence of microplastics would have negligible effects on the population dynamics of the predator-prey system (Huang and others, 2020).
2.3.1.5. Ecosystem Effects
Ultimately, the effects of microplastics on microbes and other organisms may lead to changes in ecosystem functions and services, such as productivity, nutrient cycling, carbon sequestration, and pollination (Helmberger and others, 2020; Ma and others, 2020). As a result, scientists have used multiple lines of research to investigate the effect of microplastics on ecosystems. For productivity, a large-scale model of the North Sea (lat 56.5110° N., long 3.5156° E.) predicted that microplastics would not affect the total primary or secondary production, but spatial patterns of secondary production would be altered (Troost and others, 2018). Nutrient cycling could be affected by microplastics by providing a novel substrate for colonization by a plastic-specific assemblage of microbes (Eckert and others, 2018; Oberbeckmann and others, 2018; Chen and others, 2020) and by altering filtration rates by benthic bivalves (Cluzard and others, 2015; Green and others, 2017). In aquatic ecosystems, microplastic biofilms accelerate ammonia and nitrite oxidation as well as denitrification (Chen and others, 2020). For bivalves, filtering rates of Ostrea (oysters) increased in the presence of microplastics, leading to reduced porewater ammonium concentrations and biomass of benthic cyanobacteria (Green and others, 2017). On the other hand, Mytilus (mussel) filtering rates did not change in the presence of microplastics (Green and others, 2017), while ammonium concentrations increased in the water surrounding commercial clams contaminated with microplastic beads (Green and others, 2017). Green and others, (2017) implied that their results showing increased ammonium concentrations in the water around clams exposed to microplastics could potentially lead to increased algal blooms around commercial shellfish operations. In terrestrial ecosystems, microplastics increased nutrient contents of organic matter dissolved from soil (Liu and others, 2017). Finally, a review of the effects of microplastics on insects indicated that multiple ecosystem services provided by insects could be affected including pollination, mostly because microplastic beads could mimic pollen (Oliveira and others, 2019). Interestingly, Oliveira and others (2019) also indicated that certain insect larvae might be able to help remediate the effects of plastics by consuming and digesting them.
2.3.2. Science Gaps
There are many gaps in our understanding of the acute and chronic toxicity of microplastics on individuals, populations, and ecosystems. Teasing out the complex interactions of microplastic particles, intrinsic and adsorbed compounds, and other environmental stressors is necessary, but difficult, especially in wild populations. Microplastics must be considered in conjunction with the other chemical contaminants and biological stressors, such as pathogens and parasites. Additionally, for cold-blooded aquatic organisms such as invertebrates and fishes, metabolic and immune responses and reproduction are affected by climatic factors such as temperature and photoperiod that must be considered. Most of the available aquatic vertebrate toxicology data come from laboratory studies of model species, such as zebrafish (Bhagat and others, 2020). These model species are useful as they are easily cultured, small, easily bred and maintained, and have a short life cycle and well-documented genetics. However, questions have been raised about the relatively high concentrations of microplastics used in laboratory exposures and application of the findings to longer lived species (Roch and others, 2020). Researchers are quick to point out that concentrations in the environment are too low to elicit many of the effects shown in the laboratory (de Ruijter and others, 2020) and many of the effects (such as growth, immune response, reproduction, and tissue inflammation) seen in the laboratory are not uniquely attributable to plastic particles. Similar effects can occur due to naturally occurring particles and other confounding factors (Ogonowski and others, 2018; Roman and others, 2021). Identification of sensitive species, effects on economics and threatened and endangered species, and species most closely tied to bioaccumulation through food webs is necessary.
2.3.3. USGS Capabilities and Expertise
The USGS has a long history of research in ecotoxicology and ecosystem science and is well positioned to tackle the science gaps involving the toxic effects of microplastics on organisms, communities, and ecosystems. The infrastructure and personnel for creating large-scale controlled experiments and landscape-level field studies of organismal and ecosystem responses to chemical contaminants have been well demonstrated within multiple disciplines and mission areas (for example, ecosystems, water resources, and energy and minerals). The USGS has multiple laboratory facilities to complete ecotoxicological studies. Some of these aquatic laboratory systems were designed to remove contaminants from the effluent stream and include physical filtration systems sufficient to remove microplastics. Other laboratories designed for mesocosm studies (for a range of species, including invertebrates, fish, mussels, amphibians, birds, and mammals) can be simply retrofitted for this same removal capability. In addition to these facilities, the USGS has many pond facilities, living streams, and specialized containment laboratories that could be retrofitted for contaminant removal processes and for studies to answer questions concerning microplastics and relevant pathogens. These laboratories are dispersed across the Nation and are run by a diverse network of staff who are capable for deployment for both field- and laboratory-related studies following a structured and documented Quality Management System that ensures quality in work processes, products, and laboratory services. The Quality Management System was revised in 2022 (U.S. Geological Survey, 2022).
2.3.4. USGS Science Opportunities
2.3.4.1. Short-Term Opportunities
The USGS could prioritize toxicological studies with different plastic polymers by (1) using data from new studies, (2) identifying the relative likelihood of toxicity of different polymers and associated chemicals by consulting existing databases such as CompTox (Williams and others, 2017) and ToMEx (Thornton Hampton and others, 2022), and (3) identifying sensitive and economically important species. Examples of species to study include Atlantic and Pacific herring (Clupea spp.) or river herring (Alosa spp.), anchovies (family Engrulidae), American eel (Anguilla rostrata), shrimp (family Caridae), and red snapper (Lutjanus campechanus). Integrative studies could prioritize toxicological studies within the USGS from multiple disciplines and within multiple mission areas, including ecosystems, water resources, and energy and minerals. This prioritization would start the transition from microplastics being generally considered as a single contaminant to categorizing and defining microplastics by toxicologically relevant groups. After prioritization, studies could focus on acute and chronic toxicity tests measuring sublethal endpoints in sensitive species across trophic levels (microbes to secondary consumers). Toxicity studies should consider different exposure routes and potential mixtures while being designed for use in environmental risk assessment applications (well replicated over a range of concentrations for the development of dose-response curves). Polymer-specific toxicity testing methods should be developed for many polymer morphologies (types) and sizes and should reflect what is most prevalent in environmental occurrence studies (fibers and nanoplastics). For toxicity modeling and prediction, sorption constants of exogenous and intrinsic chemicals could be determined for chemicals on a polymer-specific basis (table 3).
2.3.4.2. Long-Term Opportunities
Experimental approaches for understanding microplastic toxicity need to be identified to better characterize microplastic toxicity with both physical and chemical modes of action. Studies are needed to understand the toxicity of both virgin and weathered microplastics. Once individual polymer, exogenous chemical, and polymer with intrinsic chemical studies are completed, more work will be needed on polymer mixture toxicity and sorbed chemical mixtures. Systems toxicology studies could be designed to elucidate the mechanism of toxicity of different polymers by integrating in vitro and in vivo toxicity data with computational modeling to inform and predict toxicity of microplastics to species not tested. Lastly, microcosm and mesocosm studies, completed alongside field studies, could be used to confirm laboratory effects in natural systems and to test microplastics' effects on populations and at the ecosystem level. Field studies, in particular, could be used to reveal the ecological and ecosystem effects of microplastics on patterns and processes such as decomposition, insect emergence, nutrient cycling, predator-prey interactions, and biomass and distribution of species of interest.
Table 3.
Short- and long-term science opportunities for researching the ecotoxicology of microplastics.[Short- and long-term science opportunities determined through literature reviews and gap analyses. USGS, U.S. Geological Survey]
2.4. Sampling Protocols
Environmental sample collection for microplastics in water, on land, in air, and in biota has been increasing substantially over the past decade; however, sample collection methods vary widely. Standard sample collection protocols are especially lacking for collection of microplastics in surface water and groundwater. Developing standardized sampling protocols is critical for data usability and transferability between sites and projects (Watkins and others, 2021; Weis and Palmquist, 2021). While the objectives of the study dictate the way in which samples are collected, inherent biases exist in the two most common water-sampling techniques—surface skimming and grab sampling—that limit the ability to extrapolate throughout the water column or across a waterbody. Several recent critical reviews summarized the various methods for surface-water sampling and offered suggestions for standardization (He and others, 2018; Koelmans and others, 2019; Prata and others, 2019a; Watkins and others, 2021). In late 2020, ASTM International (formerly American Society for Testing and Materials), in coordination with international partner organizations, drafted a standard practice for collecting water samples for identifying and quantifying microplastics in water that relies on pumping and field fractionation rather than net skimming (ASTM International, 2020). Regardless of the collection method, precautions should be taken to minimize microplastic contamination from sampling equipment and containers, clothing, processing surfaces, and the atmosphere (Brander and others, 2020; Scopetani and others, 2020).
2.4.1. State of the Science
Of all media types, surface waters have been the most studied for microplastics, with the number of samples collected in marine waters outnumbering those collected in freshwater and inland systems (Akdogan and Guven, 2019). Variability in sampling methods is the result of available equipment and study objectives. Neuston, plankton, or (more recently) microplastics nets with a rectangular frame and long sock to trap floatables in the water are the traditional ways in which samples are collected, with concentrations calculated based on the measured volume of water that passed through the net. Many researchers have used a standard net mesh size of 333 μm (Baldwin and others, 2016; Lenaker and others, 2019; Coles and others, 2020), even though some have used mesh sizes as small as 100 μm (Baldwin and others, 2020). Expected ranges in turbidity and sample conditions (for example, the amounts of algae, leaves, and other organic materials in the water) are important considerations during net selection to govern the amount of water that passes before clogging. Surface net samples tend to bias toward more buoyant particles that may not exist throughout the water column, and several studies, including one by the USGS (Lenaker and others, 2019), have evaluated the effectiveness of sampling with smaller nets at multiple depths to supplement surface skimming (Dris and others, 2018). Representative sampling is critical to thoroughly define the composition of plastic particles in the water column: vertical partitioning is affected by polymer density, and sampling only the water surface will bias results toward low-density polymers and inaccurate concentrations and loads (Lenaker and others, 2019). Field replicates and blanks of sampling equipment are commonly collected to assess variability and bias in sampling routines, including potential contamination from sampling nets and other plastic equipment.
Whole-water surface-water samples have been used for quantifying microplastics and typically require collection of large volumes of water that are transported to a laboratory for filtration. These samples generally result in a small fraction of the volume represented compared to in situ net sampling, resulting in less reproducible counts than those for samples collected with nets (ASTM International, 2020). The ASTM International water sampling method for identification and quantification of microplastics is analogous to a point sample collected within a representative part of a waterway or effluent stream. However, the USGS is experimenting with a modified version of the method that is a combination of pumping cross-sectional points in the water column (similar to equal-depth increment or equal-width increment sampling [U.S. Geological Survey, 2006]) and passing water through a stack of sieves in the field at a larger volume (>1,000 liters [L]) than typical grab sampling can provide.
There is limited research on microplastic contamination in groundwater. Studies of springs, groundwater wells, and drinking water supplies have reported a predominance of microplastic fibers relative to other morphologies (Alimi and others, 2018; Koelmans and others, 2019; Panno and others, 2019). Agricultural drainage water has been identified as a source of microplastics in shallow groundwater (Bigalke and others, 2022). Aquifer material (for example, karst versus glacial unconsolidated sediment) and the overlying composition and thickness of the vadose (or unsaturated) zone all play a role in determining the extent to which groundwater can become contaminated from land and shallow subsurface sources (Alimi and others, 2018). Microplastic concentrations in groundwater are typically lower than those in surface waters; therefore, a collection of larger sample volume is recommended (Koelmans and others, 2019). Because groundwater typically contains much less organic matter and sediment than surface waters, large volumes (>1,000 L) of whole-water surface-water samples can be collected and filtered without the risk of clogging. The potential for contamination during transportation and processing meant that passing groundwater and (or) drinking water through a sieve or filter at the point of collection has been preferred (Koelmans and others, 2019).
Sample collection for microplastics in soils, bed sediment, and biosolids resembles more traditional approaches for chemical and microbiological assessments (Corradini and others, 2019; Prata and others, 2019b; Corcoran and others, 2020). Since about 2013, samples of bed sediments have been collected from lakes, beaches, rivers, and some of the deepest known marine environments (Woodall and others, 2014; Whitmire and Bloem, 2017; Barrett and others, 2020). Bottom material and shoreline sediment represent a sink for microplastics and, thus, can contain high concentrations relative to the adjacent water. Surficial sediment can be collected by shovel and (or) scoop in terrestrial environments or different forms of grab samplers such as a ponar in aquatic environments (Prata and others, 2019a). Core samples have also been collected to provide a timeline of microplastic deposition (Turner and others, 2019; Baldwin and others, 2020). Sample collections at wastewater treatment facilities and agricultural fields have mainly been focused on determining overall removal efficiency and loading to fields, respectively, and required only a small sample volume because of the high concentrations of microplastics in these waste media (Ziajahromi and others, 2021).
Atmospheric deposition sample collection focuses on two general types of deposition: wet, driven by rain or snow, and dry (that is, dust) deposition in the absence of wet precipitation. Recently, Brahney and others (2020a) completed a study of wet and dry atmospheric deposition of microplastics in the western United States in which they reported that 98 percent of samples contained at least some microplastics. Dry atmospheric deposition sampling for microplastics has typically followed Dossett and Bowersox (2019); however, recent publications have adapted the standard dry deposition sampling chamber with a custom-built dry sampling unit typically used for dust collection called a Brahney bucket (Brahney and others, 2020b). Collection of atmospheric deposition quality-control field samples has followed standard National Atmospheric Deposition Program/National Trends Network field quality-assurance protocols (Brahney and others, 2020a; National Atmospheric Deposition Program, 2022).
Procedures for the collection of biological samples for microplastic analysis generally follow methods used for other contaminants (Wang and others, 2017; Hermsen and others, 2018; Wang and Wang, 2018; Xu and others, 2020) with additional precautions to minimize microplastic contamination (Brander and others, 2020; Scopetani and others, 2020). Several studies have sampled microplastics by purchasing fish directly from fisherman on docks or in fish markets, which may be a relevant method if the study focus is on human health and human consumption of microplastics (Jabeen and others, 2017; Li and others, 2018), but not on natural populations (Hermsen and others, 2018).
As a leading Federal agency in scientific environmental data, the USGS can work with university researchers and other Federal agencies to develop nationally consistent sampling protocols for microplastics in surface water, groundwater, sediment, soil, air, and biota that provide representative environmental samples to inform decision makers and modeling efforts.
2.4.2. Science Gaps
2.4.2.1. Sampling Protocols
The need for standard protocols for collection of environmental microplastic samples is widely recognized as a critical obstacle in the science of microplastics (Koelmans and others, 2019; Brander and others, 2020; Watkins and others, 2021; Gimiliani and Izar, 2022). This is especially true for collection of microplastics in surface water. As of 2023, meaningful comparison of data across studies of microplastics in water is hindered by inconsistent sample collection methods and analyses (Hermsen and others, 2018), which have evolved along with the understanding of environmental occurrence, the potential for sample contamination, and improved analytical techniques, among other factors. Thus, there is a need for a comprehensive assessment of existing sampling protocols, especially in water, to develop a set of standardized methods that can be used to collect comparable data by personnel across the United States and in a range of conditions. The recent development of a sampling method by ASTM International (ASTM International, 2020) is a significant step toward standardization and could improve interstudy comparability among USGS studies. Before using any method, USGS laboratories are required to verify they can perform outside methods while meeting established acceptance criteria or will have to validate the suitability and performance of any newly developed methods (U.S. Geological Survey, 2022). Practical considerations, such as the amount of time and the equipment and supplies required to collect a sample, should also be considered.
2.4.2.2. Quality Control
Quality-control considerations unique to microplastics need to be included in the development of standardized microplastic sampling protocols. Perhaps the most important consideration is the potential for contamination. Because microplastics come from a wide variety of sources and are ubiquitous in both indoor and outdoor environments, there is a high potential for contamination during all stages of sample collection, processing, and analysis (Brander and others, 2020). Equipment, field, and laboratory blanks are therefore critical to assess potential contamination throughout the sample collection and analysis process. Replicate samples are also necessary to understand microplastic heterogeneity and sample representativeness. A quality-control consideration unique to microplastics is that microplastic densities vary by polymer, and therefore particles of different polymer types will distribute differently in the water column and sediment (Lenaker and others, 2019). Although not considered in many microplastic studies, this vertical heterogeneity is an important consideration in minimizing bias and collecting vertically representative samples in water. In studies to date, quality-control efforts related to sample collection have been inconsistent (Koelmans and others, 2019). Thus, to help guide the development of robust sample-collection methods, there is a need for rigorous assessment of quality assurance related to (1) maximizing sample representativeness and reproducibility in different media, (2) minimizing bias, and (3) minimizing contamination.
2.4.3. USGS Capabilities and Expertise
Among USGS microplastic studies, an effort has been made to use consistent sampling methods, but changes have been implemented over time to reflect current (2023) best practices and to meet specific study needs. To date, most USGS studies involving surface water sampling of microplastics have used the same protocols outlined by Baldwin and others (2016), but they have varied in their use of net mesh sizes, net floatation devices, sampling depths, and net rinsing procedures (Baldwin and others, 2016; Lenaker and others, 2019; Baldwin and others, 2020; Coles and others, 2020). USGS studies of atmospheric deposition of microplastics (Wetherbee and others, 2019) have followed the protocols of the National Atmospheric Deposition Program (Dossett and Bowersox, 2019), using a combination wet/dry collector equipped with a precipitation sensor and a mobile sample-chamber cover. Sample collection and analysis of microplastics in bed sediment (Baldwin and others, 2020, 2021; Lenaker and others, 2021), soil (per D.W. Kolpin, USGS and coauthor of this report, written commun., 2020), and biota (Spanjer and others, 2020; Baldwin and others, 2020, 2021) by USGS researchers have been specific to project needs. Sample collection methods have evolved over time, complicating the comparison of data across studies and highlighting the need for standardized sample collection procedures for each of the media.
The USGS has a long history of developing protocols for sampling organic and inorganic constituents in water, sediment, biota, and air, and it has the facilities and expertise to address many of the existing gaps related to the collection of microplastic samples. The resources include the USGS National Water-Quality Network, along with an array of hydrologic research laboratories stationed around the Nation. The most critical gaps among the different types of media are in standard sampling protocols for surface water (including stormwater) and groundwater. These critical gaps in sampling protocols can be filled from multiple disciplines and within multiple USGS mission areas (for example, ecosystems, water resources, and energy and minerals). University researchers and Federal partners (EPA, NOAA, NIST, and USGS) have already taken steps toward standardizing metrics and sampling protocols (Masura and others, 2015; U.S. Environmental Protection Agency, 2017; Cowger and others, 2020). It is therefore imperative that the USGS continue to work within these partnerships to develop consistent sampling protocols across agencies and ensure that USGS research remains relevant.
2.4.4. USGS Science Opportunities
2.4.4.1. Short-Term Opportunities
In the short term (1–2 years), the USGS could use its expertise and existing laboratory capabilities (see section 2.5.3., “USGS Capabilities and Expertise”) to evaluate existing sampling protocols, with the longer term goal of developing a nationally consistent protocol for the collection of microplastics samples in water (like surface water, stormwater, groundwater, drinking water), bed and suspended sediment, soils, and biota (table 4). As an example, the USGS could use the flumes at its hydraulic laboratory (Hydrologic Instrumentation Facility) to test different water column microplastics sampling methods under varying flow conditions and identify the strengths, weaknesses, and biases of each method. These and other activities should be done in cooperation with researchers at other Federal agencies and universities, who have together made significant progress toward these goals.
2.4.4.2. Long-Term Opportunities
In the long term (3 or more years), the USGS could refine and publish sampling protocols for microplastics in different media, based on the evaluations described in section 2.4.4.1., “Short-Term Opportunities,” to meet the data-quality standards of the USGS and other agencies. Publication of standardized, nationally consistent sampling protocols would enable meaningful comparison of microplastic data across studies and agencies. Additionally in the long term, the USGS could partner with researchers who are developing sensors and machine learning techniques to provide real-time measurements or surrogate estimates of microplastics in surface waters.
Table 4.
Short- and long-term science opportunities for evaluating and developing sampling protocols for microplastics.[Short- and long-term science opportunities determined through literature reviews and gap analyses. USGS, U.S. Geological Survey, QA/QC, quality assurance and quality control]
2.5. Analytical Methods
2.5.1. State of the Science
Microplastic analysis is complex because of (1) the need to isolate plastic material from the sampling matrix, (2) the large number of polymers and diversity of chemical compositions, (3) the changing properties of plastics as they oxidize and degrade in the environment, and (4) adsorbed and absorbed chemicals from the aquatic and atmospheric environment (Hale, 2017). Unlike water-quality data that the USGS collects on trace-organic chemicals, data for microplastic research needs to characterize the abundance of individual particles and their sizes and morphologies, in addition to their chemical composition and concentration, (Hale, 2017). The physical state of microplastics in the environment means that researchers often must enumerate and characterize a specific size and class of a solid polymer rather than being able to measure a dissolved fraction as with other commonly analyzed contaminants. This need to count particles has presented a challenge when calculating concentrations of microplastics with current (2023) analytical tools. NOAA released the first widely accepted and used protocol in a 2015 technical memorandum (Masura and others, 2015). The NOAA visual counting method was used in many studies and was an essential first step in standardizing methods across different laboratories (commercial, government, and academic). Existing analytical methods for microplastics can generally be broken down into the following components: (1) isolation from the environmental matrix, (2) identification and quantification of physical particles, (3) chemical characterization of the particle (polymer), and (4) identification and quantification of intrinsic and exogenous chemicals accumulated by microplastics.
Analytical methods to date have relied on protocols from academic journals and, frequently, on a method developed and supported by the NOAA (Masura and others, 2015; Baldwin and others, 2016) to enumerate and categorize microplastics in water and sediment. Analytical protocols are now available through ASTM International for sample preparation (ASTM International, 2020). However, in 2023, ASTM International methods are not approved for characterization (counting or polymer identification). ASTM and EPA practices and methods in development include 67563 Collection Practice, 67564 Preparation Practice, 67565 IR and Raman Test Method, 67788 Pyrolysis-Gas Chromatography-Mass Spectrometry (Pyr-GC/MS) Test Method, and 70831 Reference Sample Fabrication Practice (Cook and Allen, 2020). To the best of the authors’ knowledge, as of 2022, California’s State Water Resources Control Board is the only government entity in the United States to officially adopt specific analytical methods (Wong and Coffin, 2021a, b) under a regulatory mandate to analyze microplastics in drinking water. Their chosen method relies on a combination of visual (microscopy) particle identification and polymer confirmation with Raman spectroscopy (for particles larger than 20 µm) or infrared spectroscopy (for particles larger than 50 µm).
In most protocols, microplastic analysis first requires the isolation and separation of polymers in a sample from the surrounding matrix before counting, weighing, and characterizing microplastics. Depending on sample type, the general steps needed can include the chemical digestion of organic material and separation of particles by density and size. The most frequently used method to remove natural organic matter from water and sediment samples is wet peroxidation (or Fenton reaction) using hydrogen peroxide and an iron catalyst (Prata and others, 2019a). Other digestion techniques include, either individually or in combination, acid (De Witte and others, 2014), alkali (for example, potassium hydroxide is most often used for tissue digestion), enzymatic (Löder and others, 2017), or Schweizer's reagent (ASTM International, 2020). These techniques vary in effectiveness depending on the complexity of the matrix and the material co-occurring within a sample. Density separation is frequently used to extract microplastics from sediments using solutions such as lithium metatungstate or zinc chloride. Wet sieving is used for microplastics to analyze specific size fractions after isolation; however, it becomes challenging for plastics smaller than 100 µm that cannot be easily handled by an analyst using forceps under magnification. For these smaller particles, advanced techniques, such as flow cytometry and fixed fractional flow, are being tested and used to classify particles by size (Sgier and others, 2016; Summers and others, 2018; Kaile and others, 2020; Primpke and others, 2020).
After separation and size fractionization, different microplastic characteristics can be measured depending on the study’s objectives. Concentrations can be reported as counts of plastic particles per unit of volume or as polymer mass per unit volume reported for different size ranges. Particles are characterized by their morphology and color in generalized functional categories, such as fibers, fragments, or foams (Masura and others, 2015). Advanced analysis can identify and determine the mass of polymer types, such as high-density polyethylene or polyester. More advanced methods report on concentrations of chemical additives, exogenous chemicals, and bacteria or pathogens adsorbed to or leaching from microplastics. Appendix 1 contains a comprehensive list of methods and their relative advantages and limitations for identification, characterization, and quantitation techniques.
Microplastic analysis has advanced since NOAA published a visual counting method (Masura and others, 2015). Today, studies that rely on visual identification and counting are being augmented with spectroscopy methods to identify plastic types using spectral databases and enumerate microplastic particles using automated imaging functions (if scanning equipped). The most frequently used technologies are infrared (such as Fourier-transform infrared spectroscopy [FTIR]) and Raman spectroscopy (Elkhatib and Oyanedel-Craver, 2020) on single particles or on entire filter surfaces with automated scanning arrays. Automated identification can be achieved using image-analysis software with optical microscopes and with Fourier-transform infrared spectroscopy coupled with microscopy (micro-FTIR) imaging, which combines an optical microscope with FTIR in the focal plane array mode (Dris and others, 2015; Dyachenko and others, 2017; Primpke and others, 2017; Elkhatib and Oyanedel-Craver, 2020). In addition to spectral techniques, chemical analysis after thermally decomposing (burning) a sample via pyrolysis-gas chromatography-mass spectrometry (Pyr-GC/MS) can report the microplastic content of a sample as a polymer mass per unit volume (Fischer and Scholz-Böttcher, 2019; Kirstein and others, 2021). Pyr-GC/MS holds promise to characterize microplastic pollution levels more quickly but does not provide information on the number of particles in a sample. These three technologies—FTIR, Raman, and Pyr-GC/MS—are being used together and separately to answer many of the questions driving microplastic research, including sources, occurrence, and fate. Validated methods using any technology are still developing and are likely to change substantially in the coming years. Unlike standard environmental chemical analyses of organic and metal compounds, there are significant barriers due to the lack of standard quality-assurance measures.
Quality assurance and quality control (QA/QC) measures in laboratories are vital to confirm the analytical consistency, accuracy, and precision of the analyte of interest. Microplastic analysis is hampered by a lack of quality-assurance materials, protocols, and interlaboratory comparisons; the absence of these features complicate the choice of a robust and reproducible analytical method (Silva and others, 2018a; Brander and others, 2020; Ziajahromi and Leusch, 2022). One of the concerns is the lack of commercially available standard reference materials (SRMs) that represent the diverse number of polymers and their varied weathering conditions. In 2023, many studies use self-made SRMs to spike samples and calculate analytical recoveries (Gouin and others, 2019). NIST is working toward developing microplastic SRMs for better quality control (Lynch, 2019) and the Center for Marine Debris Research at the University of Hawai'i is, as of this writing (2023), selling a polymer kit that includes 22 different polymer types with standard sizes and chemical compositions. While important to accurately check spectroscopy performance, compare data across laboratories, and confirm analytical recoveries, the 22 represented polymers (as of 2023) are a small fraction of the range of polymers in the environment. As analytical methods advance, using SRMs and analytical recovery spikes in the laboratory and incorporating field and method blanks will improve data quality and increase the demand for high-quality, certified, and commercially available standards.
In addition to optical microscopy and spectral methods, several specialized analytical techniques are being investigated and used for microplastic analysis. Staining techniques, such as Nile Red, enable researchers to enumerate plastics using simple microscope cameras and image analysis software (Shim and others, 2016; Erni-Cassola and others, 2017; Maes and others, 2017). Flow cytometry (with and without fluorescence), high-temperature gel-permeation chromatography, quantitative nuclear magnetic resonance spectroscopy, laser direct infrared spectroscopy, and scanning electron microscopy are the other technologies covered in appendix 1. For a variety of reasons, whether cost, sample preparation requirements, familiarity, ease of use, or machine accessibility, these techniques have yet to gain wide acceptance and adoption by practitioners in the field.
While plastic identification and particle counting techniques are essential, it is also necessary to identify the adsorbed pollutants and study their fate and effects. Microplastics can be vectors of toxic chemicals intrinsic to their production, such as plasticizers, antioxidants, dyes, and heavy metals (Talsness and others, 2009; Brennecke and others, 2016; Rochman and others, 2019; Turner and others, 2020). Properties of microplastics such as size, surface area, chemical composition, and surface chemistry have caused significant scientific concerns with research scientists and the public over the potential for chemical sorption, transport, and concentration of exogenous environmental pollutants, bacteria, and pathogens (Arias-Andres and others, 2018; Silva and others, 2018b; Imran and others, 2019; Rochman and others, 2019; Pham and others, 2021; Wang and others, 2021c). Microplastics can desorb or leach both the adsorbed pollutants and chemical additives, and the different surface characteristics of plastics affect how they interact with the surrounding environmental matrix (Silva and others, 2018b). The metal leachates and endocrine-disrupting nature of the plasticizer chemicals and other sorbed organic pollutants may threaten environmental and organism health (Bakir and others, 2014a, b).
Once pollutants are desorbed or extracted from the microplastics in samples, the analytical methods used to characterize these extracts will depend on the intrinsic or extrinsic chemicals of interest. The most frequently applied analytical methods for analyzing chemicals accumulated in microplastics are gas chromatography-mass spectrometry and liquid chromatography-mass spectrometry (LC/MS) because of the high sensitivity and specificity for organic contaminants (Hong and others, 2017; Shim and others, 2017; Silva and others, 2018a). Techniques used for identification of accumulated trace metals (for example, Al, Cr, Mn, Ir, Co, Ni, Xn, Cd, and Pb), following an aqua regia (HCl:HNO3 at 3:1) extraction, could include scanning electron microscopy and energy dispersive X-ray spectroscopy, inductively coupled plasma-mass spectrometry (Rochman and others, 2014), inductively coupled plasma-optimal emission spectrometry (Turner and Holmes, 2015; Turner and others, 2020), and flame atomic absorption spectrometry (Brennecke and others, 2016).
2.5.2. Science Gaps
Standardized, validated laboratory methods to measure many different types and sizes of microplastics in a variety of environmental samples such as solids (for example, soil, bed sediment, and dust), biota and biological material, water, air, food, biosolids, and other matrices are needed to create reproducible datasets with the same analytes and detection limits for comparison across studies. Improvements in separation techniques are needed to reduce inconsistent results from incomplete organic material digestion, unwanted microplastic degradation/partial oxidation, and difficult to control digestion reactions. Characterization methods should be validated across the vast array of different polymers, sizes, and conditions (weathering and aging) of microplastics. SRMs relevant to field-derived microplastics are needed to calibrate analytical instruments and validate recovery rates. Although these are not yet available, NIST has a working group (Materials Measurement Science Division’s Microplastic and Nanoplastic Metrology; https://www.nist.gov/programs-projects/microplastic-and-nanoplastic-metrology) developing SRMs to be used in microplastic research. Once consistent standards are available, a concurrent increase in routine interlaboratory comparisons and performance audits is needed to lend credibility to analytical methods. Techniques are needed to characterize small microplastics (< 20 μm) and nanoplastics (< 1 μm) that are quickly being recognized as having a greater risk to environmental health (Lei and others, 2018). Additionally, cost-effective analyses for field collected samples are needed that can rapidly assess relative microplastic contamination over space and time that can lead to targeted studies in areas with high levels of microplastics.
Additional work is needed to define which metrics (count, size, mass, polymer type, or chemical composition) are most relevant for questions of occurrence, fate, and toxicity of microplastics. These metrics require different analytical techniques, each with their own data requirements and quality-assurance considerations. Furthermore, it is unclear if current bin sizes are correctly matched to meaningful characterization (for example, potential for toxicity or ingestion), and further work is needed to inform size ranges. Size bins are qualitative at best when using physical separation protocols like sequential wet sieving or filtering to separate into size fractions (Hidalgo-Ruz and others, 2012) due to interference by organic materials in the sample (for example, adhesion of small plastic fibers to larger sticks) and inherent variation of size distribution among polymer types; that is, fibers thousands of microns long may be thin enough to pass through smaller sieve sizes, or fibers can clump together and (or) break into smaller particles during processing. Improved size characterization techniques, such as flow cytometry, should be prioritized. Finally, standard protocols and methods are needed to measure the diverse array of adsorbed pollutants and chemical additives associated with microplastics, which can be interfered with by standard oxidation techniques to remove organic material.
There is also a need for continued research into developing in situ analysis of water and sediment for direct measure of microplastics and developing surrogates for estimating microplastics in real time. Although this is an ambitious goal, technological advancements being made in optical and electrode sensors (Shimizu and others, 2017; Turner, 2017; Asamoah and others, 2019; Peiponen and others, 2020) and machine learning or artificial intelligence (Zhu and others, 2021) may make future field quantification possible.
2.5.3. USGS Capabilities and Expertise
The USGS is well positioned and has the capability to address many of the science gaps, having a long history of characterizing novel and emerging contaminants of concern in water, sediment, soils, and biological samples. The USGS will need to build capabilities and capacities in a combination of microplastic measurement techniques (1) by working internally across mission areas (for example, ecosystem, water resources, and energy and minerals) and (2) through partnerships with academic institutions, contract laboratories, and other Federal agencies. To develop these analytical capabilities, the USGS can use the skills of research scientists at our many laboratories across the United States. Within the last couple years, the USGS has made strategic investments in analytical instruments, such as Raman or laser direct infrared imaging, to align USGS analytical methods with those used by other Federal agencies and academic institutions. The USGS can also expand applications of the existing instrumentation and workforce to develop high throughput methods (such as fluorescent flow cytometry and LC/MS), advance methods related to the characterization of adsorbed and exogenous chemicals, and participate in interlaboratory comparison studies. Other development and standardization of complementary analytical techniques should be fit-for-purpose, with study and stakeholder needs guiding decisions on workforce training, capital investments, and external collaboration.
2.5.4. USGS Science Opportunities
2.5.4.1. Short-Term Opportunities
In 2023, the USGS can use existing mass spectrometry expertise, equipment, and techniques, such as gas chromatography-mass spectrometry, LC/MS, and inductively coupled plasma-mass spectrometry, for analyzing microplastic matrices (table 5). These techniques are currently used for contaminant analyses (for example, with mercury, per- and polyfluoroalkyl substances, and polychlorinated biphenyls). This equipment could be immediately put to use in the characterization of sorbed intrinsic and extrinsic compounds (organic and inorganic contaminants) and the role of microplastics in fate, exposure, and adverse effects of these sorbed chemicals. Investment in new mass spectrometry techniques like Pyr-GC/MS or thermal desorption gas chromatography-mass spectrometry (TD-GC/MS) would provide complementary chemical composition information, and quantification of mass of different microplastic polymer types, with better reproducibility than optical methods. The mass spectrometric determination allows microplastic quantities to be presented in traditional concentration units (mass per unit volume), which facilitates more accurate comparisons of pollution loading among polymer types. These data help with assessing source apportionment trends and allow better modeling of chemical sorption using known polymer chemical sorption kinetics.
2.5.4.2. Long-Term Opportunities
The USGS could develop, validate, and standardize methods for studying microplastics, and it could devise best practices for analyses for freshwater, wastewater, sludge, sediment, biological samples, and soil media (table 5). One capability for the USGS is the monitoring of microplastics (<300 µm) contributing to the aquatic load and potential effects on freshwater systems, which requires tools that are fast, easily implemented, and capable of detecting small microplastics. Plastic particles are known to undergo continuous fragmentation when exposed to environmental stressors (photodegradation, thermal degradation, and biodegradation), so the number of particles is expected to increase steeply for smaller sizes (Cózar and others, 2014; Andrady, 2017). Despite smaller microplastics making up the largest relative percentage of the aquatic load, these smaller fractions of <300 µm, and especially those <20 µm, they are consistently neglected in most quantification studies because large mesh neuston nets and low resolution FTIR spectroscopy (or optical microscopes) are commonly used for particle counting (Araújo and others, 2018; Conkle and others, 2018; Enders and others, 2015). Raman microscopy capabilities would offer the best opportunity for the USGS to provide accurate quantification of aquatic load in the full microplastics size range from 1 to 5 mm, along with the identification of the polymer type and associated source materials and locations (Käppler and others, 2016; Elert and others, 2017; Ivleva and others, 2017). This choice primarily stems from micro-Raman being able to identify smaller microplastics (<20 µm) that are undetectable by micro-FTIR techniques, along with its known capabilities for improving sample analysis times through automated particle counting and machine learning techniques.
Additional opportunities exist for development of in situ and real-time quantitation techniques for microplastics and (or) their accumulated toxic chemicals in water and sediment using surrogate (proxy) measurements or advancing portable technologies. Important opportunities exist due to recent technological advances in portable measuring devices with X-ray fluorescence, optical laser sensors, and microparticle-electrode sensors that can be coupled with machine learning automation in the future (Shimizu and others, 2017; Turner, 2017; Asamoah and others, 2019; Peiponen and others, 2020). The USGS is uniquely positioned with expertise in these fields to develop and implement advancements to improve microplastic field capabilities.
Table 5.
Short- and long-term science opportunities for developing standardized analytical methods to study microplastics.[Short- and long-term science opportunities determined through literature reviews and gap analyses. USGS, U.S. Geological Survey]
3.0. Vision for Interdisciplinary Science
In this section, topics from the preceding sections are integrated into a larger vision that leverages the broad interdisciplinary expertise, tools, capabilities, and field and laboratory capacities across the Nation that are unique to the USGS. The vision for interdisciplinary science relies on the continued collaborative capabilities and efforts of the diverse scientific experts and laboratory personnel across the USGS.
3.1. Interdisciplinary Science at the USGS To Support Microplastic Research
USGS interdisciplinary science focused on microplastic research is envisioned to provide a comprehensive understanding of how microplastics enter the environment, sorb pathogens and chemicals (referred to broadly as “contaminants”), and transform and move through ecosystems. (Note: Pathogens and chemicals, for the purposes of this document, refer to any physical, chemical, biological, or radiological substance or matter that has a negative effect on the environment. As stated, for the remainder of this section, these substances will simply be referred to as “contaminants.”). An interdisciplinary science approach will also provide information on pathways of exposure and health effects for both wildlife and people.
3.2. A One Health Integrated Science Approach With a Source-to-Receptor Framework
The USGS can provide data and understanding of microplastics using a One Health approach that incorporates a source-to-receptor framework (sidebar 5). The source-to-receptor approach includes interdisciplinary science to understand the environmental sources, the movement and transformation, exposure pathways, and ecotoxicity of microplastics and the chemicals that may be adhered to them. Most importantly, this approach identifies the sequence of events that must occur for there to be a health outcome, including the contaminant source, transport, exposure routes, and biological uptake (fig. 2). A One Health approach is driven by the principle that the health of humans, plants, animals and ecosystems are intricately linked (de la Rocque and others, 2023). This information allows stakeholders to make informed decisions to prevent, mitigate, remediate, or otherwise manage the hazards brought on by exposures to contaminants. Toxicological research supported by the USGS at cellular, organismal, and population levels in a variety of wildlife species provides stakeholders with a deeper understanding of the risks, if any, associated with ecological exposures.
Sidebar 5. One Health Approach
The U.S. Geological Survey (USGS) completes surveillance and research on chemical, biological, and radiological substances that occur in the environment naturally and through human activities (broadly considered environmental contaminants). The One Health approach is driven by the principle that human health and animal health are interdependent and linked to the health of the ecosystems in which they coexist. Focusing on known or suspected contaminants that affect wildlife, the USGS partners with public health agencies to identify and assess pathways of environmental exposures to the public. This approach informs responses by public and wildlife health agencies to diseases shared between animals and people, contributes data and information on public and wildlife exposures to toxicants and pathogens in the environment, and provides research, modeling, and analysis to assist resource management decision making.
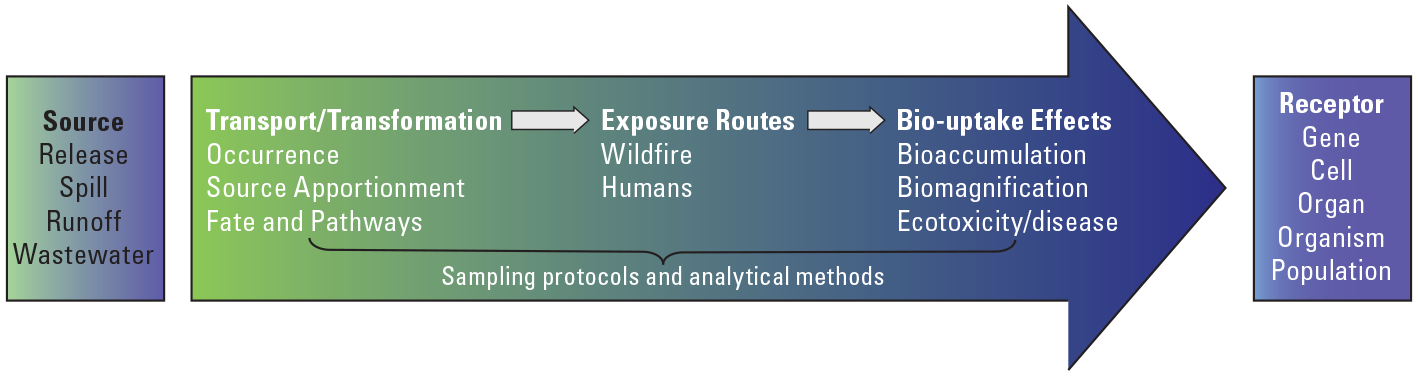
Flow chart showing the source-to-receptor framework for the study of microplastics in the environment. Critical points in this figure include: (1) source, (2) mode of transport/transformation, exposure routes, and bio-uptake effects, and (3) receptors. Examples are given for each critical point.
A One Health approach has been used in many Federal agencies and international working groups. According to the Centers for Disease Control and Prevention, One Health is gaining recognition in the United States and globally as an effective way to address health issues at the human-animal-environment interface (Centers for Disease Control and Prevention, 2023). The USGS and its partners have led interdisciplinary research efforts on an array of One Health topics, which include (but are not limited to) the effects of contaminants on aquatic ecosystems (Masoner and others, 2020; Bradley and others, 2022; Pulster and others, 2022), avian influenza (Humphreys and others, 2021; McDuie and others, 2022; Ramey and others, 2022), and climate change (Krabbenhoft and others, 2020; Stets and others, 2020; Terando and others, 2020).
The USGS has science centers throughout the Nation, with a wide range of expertise, capable of providing both laboratory and field research using rigorous techniques and methods to collect, interpret, and deliver high-quality reproducible data and analyses. Over the past few decades, there has been an increasing awareness that the health of plants, animals (including insects), and our shared environment directly affect the health of people. USGS interdisciplinary expertise in relevant disciplines such as contaminant sources, transport, and degradation, ecosystem function and health, wildlife health, geochemistry, statistics, and modeling, along with its established partnerships with other government agencies, Tribal entities, nongovernmental organizations, academia, industry, and public health entities, enables it to apply a One Health approach to studying the effects of microplastics. These collaborations are critical, as there is no single entity that can independently address all the potential health concerns associated with microplastics.
The end goal of a fully developed microplastic research implementation plan for interdisciplinary scientific studies of microplastics in the environment is beyond the scope of this strategic science vision document; this document is instead intended to provide guidance on the steps needed to move toward implementation. The conceptual approach to scientific research described in this document seeks to (1) build on existing USGS resources, scientific expertise, and capabilities, (2) provide a framework to optimize and use USGS unique resources and those resources and capabilities that are being shared among the USGS and its partners, and (3) highlight science gaps that can be addressed either by USGS science or by the USGS in collaboration with its partners to move microplastic research forward (table 6).
Table 6.
Short- and long-term science opportunities for interdisciplinary science approach to the study of microplastics.[Short- and long-term science opportunities determined through literature reviews and gap analyses. USGS, U.S. Geological Survey]
Interdisciplinary science with a One Health approach can be done on specific topics such as microplastic research, allowing attention to be focused on the entire question (the relation between the ecosystem and the health of humans and wildlife) rather than just the smaller parts, thus creating the potential for obtaining answers and informing decisions much more quickly. With this approach, both ecosystem health and economic benefit can be addressed together in an efficient manner. This information can be used by stakeholders to identify and assess pathways of environmental exposures to wildlife and the public, and it helps partners and stakeholders balance contaminant risks, if any exist, with other priorities.
3.3. Conceptual Workflow for an Interdisciplinary Science Approach to USGS Microplastic Research
The USGS is well known for its extensive geographic footprint of science centers with capabilities and expertise that span earth and natural science disciplines and include a workforce trained in nationally consistent environmental monitoring, sample collection, and laboratory analysis (sidebar 6). This expertise could be extended to include long-term (3 or more years) monitoring research projects that address current (2023) microplastic science gaps (table 6). Research concerning microplastics can be fully achieved in a One Health approach through collaborations with other government agencies, Tribal entities, nongovernmental organizations, academia, industry, and public health entities focused on ecosystem, wildlife, and human health in a true One Health partnership. The USGS can continue to provide information that helps society understand microplastic sources, pathways, and potential biological effects. The USGS and its partners will be able to answer questions such as the following: (1) Which microplastic sizes, morphologies, polymers, and additives present the greatest biological risk?, (2) How do sorbed and do co-occurring contaminants affect health risks of microplastic exposures?, (3) How can emerging technologies and sensors to monitor for microplastics and co-occurring contaminants be utilized in real time?, (4) By understanding microplastic sources, pathways, and fate can exposures be mitigated or prevented?. This information will allow the USGS to build modeling and decision tools that help anticipate the greatest threats and hazards associated with microplastics and associated contaminants. Finally, this work prioritizes areas where underserved communities may be disproportionately affected.
Sidebar 6. Vision for an Integrated Science Approach to the Study of Microplastics in the Environment
An integrated science approach will complete cross-discipline immersion on everything related to the data, techniques, tools, research, perspectives, concepts, and communication to fundamentally advance the understanding of microplastics in the environment. This information will be used to support stakeholder decisions related to the issue of microplastics in the environment on topics spanning the identification, mitigation, and prevention of any microplastic-associated hazards. The broad scope of both analytical laboratory and field expertise within the U.S. Geological Survey (USGS) can support a range of interdisciplinary and external expertise to support everything from data collection to research to publication. The wide range of studies that would include both spatial and temporal changes of microplastics across a variety of ecosystems, could provide a framework of actionable information for identifying and rectifying the most significant factors that may lead to hazards related to microplastics.
The USGS has an extremely high potential for ongoing and enhanced collaborative efforts and interdisciplinary science. To help ensure the success of interdisciplinary science on microplastics moving forward, a collaborative workflow in the USGS could be identified, developed, and implemented. Furthermore, increased collaborative effort among the USGS and other Federal, State, private industry, Tribal, nonprofit, and academic partners would be most effective to address the issues with microplastics. Although the development and implementation of these workflows is beyond the scope of this document, the critical elements of the workflow of interdisciplinary science activities within USGS include mechanisms for (1) the development of shared technical documents, (2) efficient yet effective leveraging of program resources, and (3) Bureau-level tactical plans that include monitoring for accountability (Tokranov and others, 2021). A clear and concise mode of communication and outreach is also important to coordinate and create and maintain new and existing internal networks, highlight web pages, social media, and other outlets as appropriate.
3.4. Conclusion
Contaminants can change depending on the environmental pathway, which then changes the risk of exposure and its effects. Depending on the contaminant, exposures can result in bioaccumulation and (or) immediate harm or death, or repeated exposures can lead to a range of adverse health effects (such as immune modulation, cancer, or behavioral effects) over many years or multiple generations. In other cases, there may not be any adverse health effects. The long-term effects of microplastics on the health of wildlife and humans are largely unknown. Importantly, contaminants are rarely found alone in the environment (Bradley and others, 2017). Microplastics themselves are found in complex mixtures of naturally occurring chemicals (for example, arsenic, uranium, mercury, algal toxins) and synthetic chemicals (per- and polyfluoroalkyl substances, hormones, pharmaceuticals and pesticides). The interaction of these chemicals can change how microplastics are transported through the environment and the effects they have on humans and wildlife.
The conception of this strategic science vision document, as well as all related discussions by the writing team and other colleagues at the USGS, has been instrumental in forming a vision for completing microplastic research using an interdisciplinary science approach with One Health. This approach is ideal for monitoring, modeling, and forecasting processes that drive environmental hazards related to microplastics, ecosystems and the public. This vision includes how USGS scientific expertise and capabilities can be used to address key science gaps related to microplastics in the environment and opportunities in the short and long term.
This strategic science vision provides a starting point for implementing interdisciplinary science focused on microplastics. The resulting data will (1) provide actionable information to managers at the spatially and temporally appropriate scale needed for specific decisions, (2) build coalitions of research partners, and (3) provide an information resource for scientists, resource managers, and the general public interested in microplastics research. This approach will lead to information that can be used to achieve the goal of health among people, wildlife, and the ecosystems they share. With this vision, interdisciplinary science activities can address issues at both temporal and spatial levels, as stakeholder needs evolve. Although this can be done at varying degrees of certainty with multiple workflow models, the conceptual One Health workflow provided is a robust example to support, review, and manage interdisciplinary science within USGS.
Acknowledgments
The strategic science vision presented in this report of contributions from many U.S. Geological Survey (USGS) scientists and managers in science centers from multiple mission areas across the Nation. This document was improved by thoughtful reviews from the following USGS colleagues: Dave Alvarez, Kathy Conn, Bob Joseph, Geoff Plumlee, Patty Toccalino, and Jake Weltzin.
References Cited
Ahrendt, C., Perez-Venegas, D.J., Urbina, M., Gonzalez, C., Echeveste, P., Aldana, M., Pulgar, J., and Galbán-Malagón, C., 2020, Microplastic ingestion cause intestinal lesions in the intertidal fish Girella laevifrons: Marine Pollution Bulletin, v. 151, article 110795, 6 p. [Also available at https://doi.org/10.1016/j.marpolbul.2019.110795.]
Akdogan, Z., and Guven, B., 2019, Microplastics in the environment—A critical review of current understanding and identification of future research needs: Environmental Pollution, v. 254, article 113011, 24 p. [Also available at https://doi.org/10.1016/j.envpol.2019.113011.]
Al-Jaibachi, R., Cuthbert, R.N., and Callaghan, A., 2018, Up and away—Ontogenic transference as a pathway for aerial dispersal of microplastics: Biology Letters, v. 14, no. 9, article 20180479, 4 p. [Also available at https://doi.org/10.1098/rsbl.2018.0479.]
Alimi, O.S., Farner Budarz, J., Hernandez, L.M., and Tufenkji, N., 2018, Microplastics and nanoplastics in aquatic environments—Aggregation, deposition, and enhanced contaminant transport: Environmental Science & Technology, v. 52, no. 4, p. 1704–1724. [Also available at https://doi.org/10.1021/acs.est.7b05559.]
Al-Jaibachi, R., Cuthbert, R.N., and Callaghan, A., 2019, Examining effects of ontogenic microplastic transference on Culex mosquito mortality and adult weight: Science of the Total Environment, v. 651, pt. 1, p. 871–876. [Also available at https://doi.org/10.1016/j.scitotenv.2018.09.236.]
Allen, S., Allen, D., Phoenix, V.R., Roux, G.L., Jiménez, P.D., Simonneau, A., Binet, S., and Galop, D., 2019, Atmospheric transport and deposition of microplastics in a remote mountain catchment (corrected June 18, 2019): Nature Geoscience, v. 12, no. 5, p. 339–344. [Also available at https://doi.org/10.1038/s41561-019-0335-5.]
Anbumani, S., and Kakkar, P., 2018, Ecotoxicological effects of microplastics on biota—A review: Environmental Science and Pollution Research, v. 25, no. 15, p. 14373–14396. [Also available at https://doi.org/10.1007/s11356-018-1999-x.]
Andrady, A.L., 2017, The plastic in microplastics—A review: Marine Pollution Bulletin, v. 119, no. 1, p. 12–22. [Also available at https://doi.org/10.1016/j.marpolbul.2017.01.082.]
Araújo, C.F., Nolasco, M.M., Ribeiro, A.M.P., and Ribeiro-Claro, P.J.A., 2018, Identification of microplastics using Raman spectroscopy—Latest developments and future prospects: Water Research, v. 142, p. 426–440. [Also available at https://doi.org/10.1016/j.watres.2018.05.060.]
Arias-Andres, M., Klümper, U., Rojas-Jimenez, K., and Grossart, H.P., 2018, Microplastic pollution increases gene exchange in aquatic ecosystems: Environmental Pollution, v. 237, p. 253–261. [Also available at https://doi.org/10.1016/j.envpol.2018.02.058.]
Asamoah, B.O., Kanyathare, B., Roussey, M., and Peiponen, K.-E., 2019, A prototype of a portable optical sensor for the detection of transparent and translucent microplastics in freshwater: Chemosphere, v. 231, p. 161–167. [Also available at https://doi.org/10.1016/j.chemosphere.2019.05.114.]
ASTM International, 2020, D8333–20 Standard practice for preparation of water samples with high, medium, or low suspended solids for identification and quantification of microplastic particles and fibers using Raman spectroscopy, IR spectroscopy, or pyrolysis-GC/MS: West Conshohocken, Pa., ASTM International, 4 p. [Also available at https://doi.org/10.1520/D8333-20.]
Athey, S.N., Albotra, S.D., Gordon, C.A., Monteleone, B., Seaton, P., Andrady, A.L., Taylor, A.R., and Brander, S.M., 2020, Trophic transfer of microplastics in an estuarine food chain and the effects of a sorbed legacy pollutant: Limnology and Oceanography Letters, v. 5, no. 1, p. 154–162. [Also available at https://doi.org/10.1002/lol2.10130.]
Bakir, A., Rowland, S.J., and Thompson, R.C., 2014a, Enhanced desorption of persistent organic pollutants from microplastics under simulated physiological conditions: Environmental Pollution, v. 185, p. 16–23. [Also available at https://doi.org/10.1016/j.envpol.2013.10.007.]
Bakir, A., Rowland, S.J., and Thompson, R.C., 2014b, Transport of persistent organic pollutants by microplastics in estuarine conditions: Estuarine, Coastal and Shelf Science, v. 140, p. 14–21, accessed March 22, 2024, at https://doi.org/10.1016/j.ecss.2014.01.004.
Baldwin, A.K., Corsi, S.R., and Mason, S.A., 2016, Plastic debris in 29 Great Lakes tributaries—Relations to watershed attributes and hydrology: Environmental Science & Technology, v. 50, no. 19, p. 10377–10385. [Also available at https://doi.org/10.1021/acs.est.6b02917.]
Baldwin, A.K., Spanjer, A.R., Hayhurst, B., and Hamilton, D., 2021, Microplastics in the Delaware River, northeastern United States: U.S. Geological Survey Fact Sheet 2020–3071, 4 p., accessed March 22, 2024, at https://doi.org/10.3133/fs20203071.]
Baldwin, A.K., Spanjer, A.R., Rosen, M.R., and Thom, T., 2020, Microplastics in Lake Mead National Recreation Area, USA—Occurrence and biological uptake: PLoS ONE, v. 15, no. 5, article e0228896, 20 p. [Also available at https://doi.org/10.1371/journal.pone.0228896.]
Barnes, D.K.A., Galgani, F., Thompson, R.C., and Barlaz, M., 2009, Accumulation and fragmentation of plastic debris in global environments: Philosophical Transactions of the Royal Society of London B—Biological Sciences, v. 364, no. 1526, p. 1985–1998. [Also available at https://doi.org/10.1098/rstb.2008.0205.]
Barrett, J., Chase, Z., Zhang, J., Banaszak Holl, M.M., Willis, K., Williams, A., Hardesty, B.D., and Wilcox, C., 2020, Microplastic pollution in deep-sea sediments from the great Australian bight: Frontiers in Marine Science, v. 7, article 576170. [Also available at https://doi.org/10.3389/fmars.2020.576170.]
Batel, A., Borchert, F., Reinwald, H., Erdinger, L., and Braunbeck, T., 2018, Microplastic accumulation patterns and transfer of benzo[a]pyrene to adult zebrafish (Danio rerio) gills and zebrafish embryos: Environmental Pollution, v. 235, p. 918–930. [Also available at https://doi.org/10.1016/j.envpol.2018.01.028.]
Bergmann, M., Mützel, S., Primpke, S., Tekman, M.B., Trachsel, J., and Gerdts, G., 2019, White and wonderful? Microplastics prevail in snow from the Alps to the Arctic: Science Advances, v. 5, no. 8, article eaax1157. [Also available at https://doi.org/10.1126/sciadv.aax1157.]
Bhagat, J., Zang, L., Nishimura, N., and Shimada, Y., 2020, Zebrafish—An emerging model to study microplastic and nanoplastic toxicity: Science of the Total Environment, v. 728, article 138707. [Also available at https://doi.org/10.1016/j.scitotenv.2020.138707.]
Bhagat, K., Barrios, A.C., Rajwade, K., Kumar, A., Oswald, J., Apul, O., and Perreault, F., 2022, Aging of microplastics increases their adsorption affinity towards organic contaminants: Chemosphere, v. 298, article 134238. [Also available at https://doi.org/10.1016/j.chemosphere.2022.134238.]
Bigalke, M., Fieber, M., Foetisch, A., Reynes, J., and Tollan, P., 2022, Microplastics in agricultural drainage water—A link between terrestrial and aquatic microplastic pollution: Science of the Total Environment, v. 806, no. 4, article 150709. [Also available at https://doi.org/10.1016/j.scitotenv.2021.150709.]
Bourdages, M.P.T., Provencher, J.F., Baak, J.E., Mallory, M.L., and Vermaire, J.C., 2021, Breeding seabirds as vectors of microplastics from sea to land—Evidence from colonies in Arctic Canada: Science of the Total Environment, v. 764, article 142808, accessed November 1, 2023, at https://doi.org/10.1016/j.scitotenv.2020.142808.
Botterell, Z.L.R., Beaumont, N., Dorrington, T., Steinke, M., Thompson, R.C., and Lindeque, P.K., 2019, Bioavailability and effects of microplastics on marine zooplankton—A review: Environmental Pollution, v. 245, p. 98–110. [Also available at https://doi.org/10.1016/j.envpol.2018.10.065.]
Boyero, L., López-Rojo, N., Bosch, J., Alonso, A., Correa-Araneda, F., and Pérez, J., 2020, Microplastics impair amphibian survival, body condition and function: Chemosphere, v. 244, article 125500. [Also available at https://doi.org/10.1016/j.chemosphere.2019.125500.]
Bradley, P.M., Journey, C.A., Romanok, K.M., Buxton, H.T., Jones, D.K., Foreman, W.T., Furlong, E.T., Hladik, M.L., Iwanowicz, L.R., Kolpin, D.W., Kuivila, K.M., Loftin, K.A., Mills, M.A., Meyer, M.T., Orlando, J.L., Reilly, T.J., Smalling, K.L., and Villeneuve,D.L., 2017, Expanded target-chemical assessment reveals extensive mixed-organic-contaminant exposure in U.S. streams. Environmental Science & Technology, v. 51, p. 4792–4802. [Also available at https://doi.org/10.1021/acs.est.7b00012.]
Bradley, P.M., Romanok, K.M., Smalling, K.L., Focazio, M.J., Charboneau, R., George, C.M., Navas-Acien, A., O’Leary, M., Cloud, R.R., Zacher, T., Breitmeyer, S.E., Cardon, M.C., Cuny, C.K., Ducheneaux, G., Enright, K., Evans, N., Gray, J.L., Harvey, D.E., Hladik, M.L., Kanagy, L.K., Loftin, K.A., McCleskey, R.B., Medlock-Kakaley, E.K., Meppelink, S.M., Valder, J.F., and Weiss, C.P., 2022, Tapwater exposures, effects potential, and residential risk management in Northern Plains nations: ACS ES&T Water, v. 2, no. 10, p. 1772–1788. [Also available at https://doi.org/10.1021/acsestwater.2c00293.]
Brahney, J., Hallerud, M., Heim, E., Hahnenberger, M., and Sukumaran, S., 2020a, Plastic rain in protected areas of the United States: Science, v. 368, no. 6496, p. 1257–1260. [Also available at https://doi.org/10.1126/science.aaz5819.]
Brahney, J., Mahowald, N., Prank, M., Cornwell, G., Klimont, Z., Matsui, H., and Prather, K.A., 2021, Constraining the atmospheric limb of the plastic cycle: Proceedings of the National Academy of Sciences, v. 118, no. 16, article e2020719118. [Also available at https://doi.org/10.1073/pnas.2020719118.]
Brahney, J., Wetherbee, G., Sexstone, G.A., Youngbull, C., Strong, P., and Heindel, R.C., 2020b, A new sampler for the collection and retrieval of dry dust deposition: Aeolian Research, v. 45, article 100600. [Also available at https://doi.org/10.1016/j.aeolia.2020.100600.]
Brander, S.M., Renick, V.C., Foley, M.M., Steele, C., Woo, M., Lusher, A., Carr, S., Helm, P., Box, C., Cherniak, S., Andrews, R.C., and Rochman, C.M., 2020, Sampling and quality assurance and quality control—A guide for scientists investigating the occurrence of microplastics across matrices: Applied Spectroscopy, v. 74, no. 9, p. 1099–1125. [Also available at https://doi.org/10.1177/0003702820945713.]
Brennecke, D., Duarte, B., Paiva, F., Caçador, I., and Canning-Clode, J., 2016, Microplastics as vector for heavy metal contamination from the marine environment: Estuarine, Coastal and Shelf Science, v. 178, p. 189–195. [Also available at https://doi.org/10.1016/j.ecss.2015.12.003.]
Cai, L., Wang, J., Peng, J., Tan, Z., Zhan, Z., Tan, X., and Chen, Q., 2017, Characteristic of microplastics in the atmospheric fallout from Dongguan city, China—Preliminary research and first evidence (corrected November 14, 2019): Environmental Science and Pollution Research, v. 24, no. 32, p. 24928–24935. [Also available at https://doi.org/10.1007/s11356-017-0116-x.]
California Ocean Protection Council, 2022, Statewide microplastic strategy: Ocean Protection Council website, accessed March 22, 2024, at https://www.opc.ca.gov/webmaster/ftp/pdf/agenda_items/20220223/Item_6_Exhibit_A_Statewide_Microplastics_Strategy.pdf.
Capolupo, M., Sørensen, L., Jayasena, K.D.R., Booth, A.M., and Fabbri, E., 2020, Chemical composition and ecotoxicity of plastic and car tire rubber leachates to aquatic organisms: Water Research, v. 169, article 115270. [Also available at https://doi.org/10.1016/j.watres.2019.115270.]
Carr, S.A., Liu, J., and Tesoro, A.G., 2016, Transport and fate of microplastic particles in wastewater treatment plants: Water Research, v. 91, p. 174–182. [Also available at https://doi.org/10.1016/j.watres.2016.01.002.]
Centers for Disease Control and Prevention, 2023, One Health basics: Centers for Disease Control and Prevention website, accessed May 31, 2023, at https://www.cdc.gov/onehealth/basics/index.html.
Chen, C., Chen, L., Yao, Y., Artigas, F., Huang, Q., and Zhang, W., 2019a, Organotin release from polyvinyl chloride microplastics and concurrent photodegradation in water—Impacts from salinity, dissolved organic matter, and light exposure: Environmental Science & Technology, v. 53, no. 18, p. 10741–10752. [Also available at https://doi.org/10.1021/acs.est.9b03428.]
Chen, Q., Allgeier, A., Yin, D., and Hollert, H., 2019b, Leaching of endocrine disrupting chemicals from marine microplastics and mesoplastics under common life stress conditions: Environment International, v. 130, article 104938. [Also available at https://doi.org/10.1016/j.envint.2019.104938.]
Chen, X., Chen, X., Zhao, Y., Zhou, H., Xiong, X., and Wu, C., 2020, Effects of microplastic biofilms on nutrient cycling in simulated freshwater systems: Science of the Total Environment, v. 719, article 137276. [Also available at https://doi.org/10.1016/j.scitotenv.2020.137276.]
Chen, Y., Chen, Q., Zhang, Q., Zuo, C., and Shi, H., 2022, An overview of chemical additives on (micro)plastic fibers—Occurrence, release, and health risks: Reviews of Environmental Contamination and Toxicology, v. 260, article 22. [Also available at https://doi.org/10.1007/s44169-022-00023-9.]
Chesapeake Bay Program, 2024, Plastic Pollution Action Team: Chesapeake Bay Program website, accessed January 5, 2024, at https://www.chesapeakebay.net/who/group/plastic-pollution-action-team.
Chua, E.M., Shimeta, J., Nugegoda, D., Morrison, P.D., and Clarke, B.O., 2014, Assimilation of polybrominated diphenyl ethers from microplastics by the marine amphipod, Allorchestes compressa: Environmental Science & Technology, v. 48, no. 14, p. 8127–8134. [Also available at https://doi.org/10.1021/es405717z.]
Cluzard, M., Kazmiruk, T.N., Kazmiruk, V.D., and Bendell, L.I., 2015, Intertidal concentrations of microplastics and their influence on ammonium cycling as related to the shellfish industry: Archives of Environmental Contamination and Toxicology, v. 69, p. 310–319. [Also available at https://doi.org/10.1007/s00244-015-0156-5.]
Coffin, S., Huang, G.-Y., Lee, I., and Schlenk, D., 2019, Fish and seabird gut conditions enhance desorption of estrogenic chemicals from commonly-ingested plastic items: Environmental Science & Technology, v. 53, no. 8, p. 4588–4599. [Also available at https://doi.org/10.1021/acs.est.8b07140.]
Cole, M., Lindeque, P., Fileman, E., Halsband, C., and Galloway, T.S., 2015, The impact of polystyrene microplastics on feeding, function and fecundity in the marine copepod Calanus helgolandicus: Environmental Science & Technology, v. 49, no. 2, p. 1130–1137. [Also available at https://doi.org/10.1021/es504525u.]
Cole, M., Lindeque, P., Halsband, C., and Galloway, T.S., 2011, Microplastics as contaminants in the marine environment—A review: Marine Pollution Bulletin, v. 62, no. 12, p. 2588–2597. [Also available at https://doi.org/10.1016/j.marpolbul.2011.09.025.]
Coles, J.F., Fisher, S.C., and Spanjer, A.R., 2020, Microplastics data collected from streams in the northeastern United States, 2017–2018: U.S. Geological Survey data release, accessed March 22, 2024, at https://doi.org/10.5066/P9DT5N3N.
Conkle, J.L., Del Valle, C.D.B., and Turner, J.W., 2018, Are we underestimating microplastic contamination in aquatic environments?: Environmental Management, v. 61, no. 1, p. 1–8. [Also available at https://doi.org/10.1007/s00267-017-0947-8.]
Cook, A., and Allen, H., 2020, Measuring microplastics—Building best practices & methods for collection, preparation and analysis [Presentation for Frontier Labs, January 2020]—ASTM Microplastic Method Development and Instrument Training, Koriyama, Fukishima Prefecture, Japan, January 20–23, 2020: U.S. Environmental Protection Agency, 25-slide presentation, accessed November 20, 2023, at https://cfpub.epa.gov/si/si_public_record_report.cfm?Lab=CESER&dirEntryId=348332.
Corcoran, P.L., Belontz, S.L., Ryan, K., and Walzak, M.J., 2020, Factors controlling the distribution of microplastic particles in benthic sediment of the Thames River, Canada: Environmental Science & Technology, v. 54, no. 2, p. 818–825. [Also available at https://doi.org/10.1021/acs.est.9b04896.]
Corradini, F., Meza, P., Eguiluz, R., Casado, F., Huerta-Lwanga, E., and Geissen, V., 2019, Evidence of microplastic accumulation in agricultural soils from sewage sludge disposal: Science of the Total Environment, v. 671, p. 411–420. [Also available at https://doi.org/10.1016/j.scitotenv.2019.03.368.]
Cousin, X., Batel, A., Bringer, A., Hess, S., Bégout, M.-L., and Braunbeck, T., 2020, Microplastics and sorbed contaminants—Trophic exposure in fish sensitive early life stages: Marine Environmental Research, v. 161, article 105126. [Also available at https://doi.org/10.1016/j.marenvres.2020.105126.]
Cowger, W., Booth, A.M., Hamilton, B.M., Thaysen, C., Primpke, S., Munno, K., Lusher, A.L., Dehaut, A., Vaz, V.P., Liboiron, M., Devriese, L.I., Hermabessiere, L., Rochman, C., Athey, S.N., Lynch, J.M., Frond, H. de, Gray, A., Jones, O.A.H., Brander, S., Steele, C., Moore, S., Sanchez, A., and Nel, H., 2020, Reporting guidelines to increase the reproducibility and comparability of research on microplastics (corrected October 29, 2020): Applied Spectroscopy, v. 74, no. 9, p. 1066–1077. [Also available at https://doi.org/10.1177/0003702820930292.]
Cowger, W., Gray, A.B., Guilinger, J.J., Fong, B., and Waldschläger, K., 2021, Concentration depth profiles of microplastic particles in river flow and implications for surface sampling: Environmental Science & Technology, v. 55, no. 9, p. 6032–6041. [Also available at https://doi.org/10.1021/acs.est.1c01768.]
Cózar, A., Echevarría, F., González-Gordillo, J.I., Irigoien, X., Úbeda, B., Hernández-León, S., Palma, Á.T., Navarro, S., García-de-Lomas, J., Ruiz, A., Fernández-de-Puelles, M.L., and Duarte, C.M., 2014, Plastic debris in the open ocean: Proceedings of the National Academy of Sciences, v. 111, no. 28, p. 10239–10244. [Also available at https://doi.org/10.1073/pnas.1314705111.]
Custer, C.M., Custer, T.W., Dummer, P.M., and Munney, K.L., 2003, Exposure and effects of chemical contaminants on tree swallows nesting along the Housatonic River, Berkshire County, Massachusetts, USA, 1998–2000: Environmental Toxicology and Chemistry: An International Journal, v. 22, no. 7, p. 1605–1621. [Also available athttp://https://doi.org/10.1002/etc.5620220725.]
Cuthbert, R.N., Al-Jaibachi, R., Dalu, T., Dick, J.T., and Callaghan, A., 2019, The influence of microplastics on trophic interaction strengths and oviposition preferences of dipterans: Science of the Total Environment, v. 651, p. 2420–2423. [Also available at https://doi.org/10.1016/j.scitotenv.2018.10.108.]
da Costa Araújo, A.P., and Malafaia, G., 2021, Microplastic ingestion induces behavioral disorders in mice—A preliminary study on the trophic transfer effects via tadpoles and fish: Journal of Hazardous Materials, v. 401, article 123263. [Also available at https://doi.org/10.1016/j.jhazmat.2020.123263.]
Dawson, A.L., Kawaguchi, S., King, C.K., Townsend, K.A., King, R., Huston, W.M., and Bengtson Nash, S.M., 2018, Turning microplastics into nanoplastics through digestive fragmentation by Antarctic krill: Nature Communications, v. 9, no. 1, article 1001. [Also available at https://doi.org/10.1038/s41467-018-03465-9.]
de la Rocque, S., Errecaborde, K.M.M., Belot, G., Brand, T., Shadomy, S., von Dobschuetz, S., Aguanno, R., Carron, M., Caya, F., Ding, S., Dhingra, M., Donachie, D., Gongal, G., Hoejskov, P., Ismayilova, G., Lamielle, G., Mahrous, H., Marrana, M., Nzietchueng, S., Oh, Y., Pinto, J., Rocher, X., Riviere-Cinnamond, A., Rojo, C., Scheuermann, L., Sinclair, J., Song, J., Skrypnyk, A., Traore, T., and Wongsathapornchai, K., 2023, One Health systems strengthening in countries—Tripartite tools and approaches at the human-animal-environment interface: BMJ Global Health, v. 8, no. 1, article e011236. [Also available at https://doi.org/10.1136/bmjgh-2022-011236.]
de Ruijter, V.N., Redondo-Hasselerharm, P.E., Gouin, T., and Koelmans, A.A., 2020, Quality criteria for microplastic effect studies in the context of risk assessment: Environmental Science & Technology, v. 54, no. 19, p. 11692–11705. [Also available at https://doi.org/10.1021/acs.est.0c03057.]
de Sá, L.C., Oliveira, M., Ribeiro, F., Rocha, T.L., and Futter, M.N., 2018, Studies of the effects of microplastics on aquatic organisms—What do we know and where should we focus our efforts in the future?: Science of the Total Environment, v. 645, p. 1029–1039. [Also available at https://doi.org/10.1016/j.scitotenv.2018.07.207.]
De Witte, B., Devriese, L., Bekaert, K., Hoffman, S., Vandermeersch, G., Cooreman, K., and Robbens, J., 2014, Quality assessment of the blue mussel (Mytilus edulis)—Comparison between commercial and wild types: Marine Pollution Bulletin, v. 85, no. 1, p. 146–155. [Also available at https://doi.org/10.1016/j.marpolbul.2014.06.006.]
Di, J., Reck, B.K., Miatto, A., and Graedel, T.E., 2021, United States plastics—Large flows, short lifetimes, and negligible recycling: Resources, Conservation and Recycling, v. 167, article 105440. [Also available at https://doi.org/10.1016/j.resconrec.2021.105440.]
Dossett, S.R., and Bowersox, V.C., 2019, National trends network site operation manual (ver. 3.0): Champaign, Ill., National Atmospheric Deposition Program Office, accessed August 22, 2022, at https://arlis.org/docs/vol1/123915417/opman.pdf.
Driedger, A.G.J., Dürr, H.H., Mitchell, K., and Van Cappellen, P., 2015, Plastic debris in the Laurentian Great Lakes—A review: Journal of Great Lakes Research, v. 41, no. 1, p. 9–19. [Also available at https://doi.org/10.1016/j.jglr.2014.12.020.]
Dris, R., Gasperi, J., Mirande, C., Mandin, C., Guerrouache, M., Langlois, V., and Tassin, B., 2017, A first overview of textile fibers, including microplastics, in indoor and outdoor environments: Environmental Pollution, v. 221, p. 453–458. [Also available at https://doi.org/10.1016/j.envpol.2016.12.013.]
Dris, R., Gasperi, J., Rocher, V., Saad, M., Renault, N., and Tassin, B., 2015, Microplastic contamination in an urban area—A case study in greater Paris: Environmental Chemistry, v. 12, no. 5, p. 592–599. [Also available at https://doi.org/10.1071/EN14167.]
Dris, R., Gasperi, J., Rocher, V., and Tassin, B., 2018, Synthetic and non-synthetic anthropogenic fibers in a river under the impact of Paris megacity—Sampling methodological aspects and flux estimations: Science of the Total Environment, v. 618, p. 157–164. [Also available at https://doi.org/10.1016/j.scitotenv.2017.11.009.]
Drummond, J.D., Schneidewind, U., Li, A., Hoellein, T.J., Krause, S., and Packman, A.I., 2022, Microplastic accumulation in riverbed sediment via hyporheic exchange from headwaters to mainstems: Science Advances, v. 8, no. 2, article eabi9305. [Also available at https://doi.org/10.1126/sciadv.abi9305.]
Duan, J., Bolan, N., Li, Y., Ding, S., Atugoda, T., Vithanage, M., Sarkar, B., Tsang, D.C.W., and Kirkham, M.B., 2021, Weathering of microplastics and interaction with other coexisting constituents in terrestrial and aquatic environments: Water Research, v. 196, article 117011. [Also available at https://doi.org/10.1016/j.watres.2021.117011.]
Duis, K., and Coors, A., 2016, Microplastics in the aquatic and terrestrial environment—Sources (with a specific focus on personal care products), fate and effects: Environmental Sciences Europe, v. 28, no. 1, article 2. [Also available at https://doi.org/10.1186/s12302-015-0069-y.]
Dyachenko, A., Mitchell, J., and Arsem, N., 2017, Extraction and identification of microplastic particles from secondary wastewater treatment plant (WWTP) effluent: Analytical Methods, v. 9, no. 9, p. 1412–1418. [Also available at https://doi.org/10.1039/C6AY02397E.]
Eckert, E.M., Di Cesare, A., Kettner, M.T., Arias-Andres, M., Fontaneto, D., Grossart, H.-P., and Corno, G., 2018, Microplastics increase impact of treated wastewater on freshwater microbial community: Environmental Pollution, v. 234, p. 495–502. [Also available at https://doi.org/10.1016/j.envpol.2017.11.070.]
Edo, C., Fernández-Piñas, F., and Rosal, R., 2022, Microplastics identification and quantification in the composted organic fraction of municipal solid waste: Science of the Total Environment, v. 813, article 151902. [Also available at https://doi.org/10.1016/j.scitotenv.2021.151902.]
Eerkes-Medrano, D., Thompson, R.C., and Aldridge, D.C., 2015, Microplastics in freshwater systems—A review of the emerging threats, identification of knowledge gaps and prioritisation of research needs: Water Research, v. 75, p. 63–82. [Also available at https://doi.org/10.1016/j.watres.2015.02.012.]
Elert, A.M., Becker, R., Duemichen, E., Eisentraut, P., Falkenhagen, J., Sturm, H., and Braun, U., 2017, Comparison of different methods for MP detection—What can we learn from them, and why asking the right question before measurements matters?: Environmental Pollution, v. 231, p. 1256–1264. [Also available at https://doi.org/10.1016/j.envpol.2017.08.074.]
Elkhatib, D., and Oyanedel-Craver, V., 2020, A critical review of extraction and identification methods of microplastics in wastewater and drinking water: Environmental Science & Technology, v. 54, no. 12, p. 7037–7049. [Also available at https://doi.org/10.1021/acs.est.9b06672.]
Enders, K., Lenz, R., Stedmon, C.A., and Nielsen, T.G., 2015, Abundance, size and polymer composition of marine microplastics ≥ 10 µm in the Atlantic Ocean and their modelled vertical distribution: Marine Pollution Bulletin, v. 100, no. 1, p. 70–81. [Also available at https://doi.org/10.1016/j.marpolbul.2015.09.027.]
Enyoh, C.E., Verla, A.W., Verla, E.N., Ibe, F.C., and Amaobi, C.E., 2019, Airborne microplastics—A review study on method for analysis, occurrence, movement and risks: Environmental Monitoring and Assessment, v. 191, no. 11, article 668. [Also available at https://doi.org/10.1007/s10661-019-7842-0.]
Eriksen, M., Lebreton, L.C.M., Carson, H.S., Thiel, M., Moore, C.J., Borerro, J.C., Galgani, F., Ryan, P.G., and Reisser, J., 2014, Plastic pollution in the world’s oceans—More than 5 trillion plastic pieces weighing over 250,000 tons afloat at sea: PLoS ONE, v. 9, no. 12, article e111913. [Also available at https://doi.org/10.1371/journal.pone.0111913.]
Erni-Cassola, G., Gibson, M.I., Thompson, R.C., and Christie-Oleza, J.A., 2017, Lost, but found with Nile red—A novel method for detecting and quantifying small microplastics (1 mm to 20 μm) in environmental samples: Environmental Science & Technology, v. 51, no. 23, p. 13641–13648. [Also available at https://doi.org/10.1021/acs.est.7b04512.]
Everaert, G., Cauwenberghe, L.V., Rijcke, M. de, Koelmans, A.A., Mees, J., Vandegehuchte, M., and Janssen, C.R., 2018, Risk assessment of microplastics in the ocean—Modelling approach and first conclusions: Environmental Pollution, v. 242, pt. B, p. 1930–1938. [Also available at https://doi.org/10.1016/j.envpol.2018.07.069.]
Fahrenfeld, N.L., Arbuckle-Keil, G., Beni, N.N., and Bartelt-Hunt, S.L., 2019, Source tracking microplastics in the freshwater environment: Trends in Analytical Chemistry, v. 112, p. 248–254. [Also available at https://doi.org/10.1016/j.trac.2018.11.030.]
Fischer, M., and Scholz-Böttcher, B.M., 2019, Microplastics analysis in environmental samples—Recent pyrolysis-gas chromatography-mass spectrometry method improvements to increase the reliability of mass-related data: Analytical Methods, v. 11, no. 18, p. 2489–2497. [Also available at https://doi.org/10.1039/C9AY00600A.]
Foley, C.J., Feiner, Z.S., Malinich, T.D., and Höök, T.O., 2018, A meta-analysis of the effects of exposure to microplastics on fish and aquatic invertebrates: Science of the Total Environment, v. 631–632, p. 550–559. [Also available at https://doi.org/10.1016/j.scitotenv.2018.03.046.]
Gilbreath, A., McKee, L., Shimabuku, I., Lin, D., Werbowski, L.M., Zhu, X., Grbic, J., and Rochman, C., 2019, Multiyear water quality performance and mass accumulation of PCBs, mercury, methylmercury, copper, and microplastics in a bioretention rain garden: Journal of Sustainable Water in the Built Environment, v. 5, no. 4, article 04019004. [Also available at https://doi.org/10.1061/JSWBAY.0000883.]
Gimiliani, G.T., and Izar, G., 2022, Difficulties in comparison among different microplastic studies—The inconsistency of results and lack of guide values: Environmental Toxicology and Chemistry, v. 41, no. 4, p. 820–821. [Also available at https://doi.org/10.1002/etc.5237.]
Goss, H., Jaskiel, J., and Rotjan, R., 2018, Thalassia testudinum as a potential vector for incorporating microplastics into benthic marine food webs: Marine Pollution Bulletin, v. 135, p. 1085–1089. [Also available at https://doi.org/10.1016/j.marpolbul.2018.08.024.]
Gouin, T., 2020, Toward an improved understanding of the ingestion and trophic transfer of microplastic particles—Critical review and implications for future research: Environmental Toxicology and Chemistry, v. 39, no. 6, p. 1119–1137. [Also available at https://doi.org/10.1002/etc.4718.]
Gouin, T., Becker, R.A., Collot, A.G., Davis, J.W., Howard, B., Inawaka, K., Lampi, M., Ramon, B.S., Shi, J., and Hopp, P.W., 2019, Toward the development and application of an environmental risk assessment framework for microplastic: Environmental Toxicology and Chemistry, v. 38, no. 10, p. 2087–2100. [Also available at https://doi.org/10.1002/etc.4529.]
Graham, P., Palazzo, L., Lucia, G.A. de, Telfer, T.C., Baroli, M., and Carboni, S., 2019, Microplastics uptake and egestion dynamics in Pacific oysters, Magallana gigas (Thunberg, 1793), under controlled conditions: Environmental Pollution, v. 252, pt. A, p. 742–748. [Also available at https://doi.org/10.1016/j.envpol.2019.06.002.]
Grbić, J., Helm, P., Athey, S., and Rochman, C.M., 2020, Microplastics entering northwestern Lake Ontario are diverse and linked to urban sources: Water Research, v. 174, article 115623. [Also available at https://doi.org/10.1016/j.watres.2020.115623.]
Green, D.S., Boots, B., O’Connor, N.E., and Thompson, R., 2017, Microplastics affect the ecological functioning of an important biogenic habitat: Environmental Science & Technology, v. 51, no. 1, p. 68–77. [Also available at https://doi.org/10.1021/acs.est.6b04496.]
Guo, H., Zheng, X., Luo, X., and Mai, B., 2020, Leaching of brominated flame retardants (BFRs) from BFRs-incorporated plastics in digestive fluids and the influence of bird diets: Journal of Hazardous Materials, v. 393, article 122397. [Also available at https://doi.org/10.1016/j.jhazmat.2020.122397.]
Gutow, L., Eckerlebe, A., Giménez, L., and Saborowski, R., 2016, Experimental evaluation of seaweeds as a vector for microplastics into marine food webs: Environmental Science & Technology, v. 50, no. 2, p. 915–923. [Also available at https://doi.org/10.1021/acs.est.5b02431.]
Hadiuzzaman, M., Salehi, M., and Fujiwara, T., 2022, Plastic litter fate and contaminant transport within the urban environment, photodegradation, fragmentation, and heavy metal uptake from storm runoff: Environmental Research, v. 212, pt. A, article 113183. [Also available at https://doi.org/10.1016/j.envres.2022.113183.]
Hale, R.C., 2017, Analytical challenges associated with the determination of microplastics in the environment: Analytical Methods, v. 9, no. 9, p. 1326–1327. [Also available at https://doi.org/10.1039/C7AY90015E.]
Hartmann, N.B., Hüffer, T., Thompson, R.C., Hassellöv, M., Verschoor, A., Daugaard, A.E., Rist, S., Karlsson, T., Brennholt, N., Cole, M., Herrling, M.P., Hess, M.C., Ivleva, N.P., Lusher, A.L., and Wagner, M., 2019, Are we speaking the same language? Recommendations for a definition and categorization framework for plastic debris: Environmental Science & Technology, v. 53, no. 3, p. 1039–1047, accessed March 21, 2024, at https://doi.org/10.1021/acs.est.8b05297.
Hartmann, N.B., Rist, S., Bodin, J., Jensen, L.H.S., Schmidt, S.N., Mayer, P., Meibom, A., Baun, A., 2017, Microplastics as vectors for environmental contaminants—Exploring sorption, desorption, and transfer to biota: Integrated Environmental Assessment and Management, v. 13, no. 3, p. 488–493, accessed March 22, 2024, at https://doi.org/10.1002/ieam.1904.
Hataley, E., Kidd, K., Rochman, C., and Rooney, R., 2023, Developing frameworks for monitoring and assessing the ecological risk of microplastics in the Laurentian Great Lakes: International Joint Commission website, accessed January 5, 2024, at https://www.ijc.org/en/developing-frameworks-monitoring-and-assessing-ecological-risk-microplastics-laurentian-great-lakes.
He, D., Luo, Y., Lu, S., Liu, M., Song, Y., and Lei, L., 2018, Microplastics in soils—Analytical methods, pollution characteristics and ecological risks: Trends in Analytical Chemistry, v. 109, p. 163–172. [Also available at https://doi.org/10.1016/j.trac.2018.10.006.]
Hebner, T.S., and Maurer-Jones, M.A., 2020, Characterizing microplastic size and morphology of photodegraded polymers placed in simulated moving water conditions: Environmental Science, v. 22, no. 2, p. 398–407. [Also available at https://doi.org/10.1039/C9EM00475K.]
Helmberger, M.S., Tiemann, L.K., and Grieshop, M.J., 2020, Towards an ecology of soil microplastics: Functional Ecology, v. 34, no. 3, p. 550–560. [Also available at https://doi.org/10.1111/1365-2435.13495.]
Hermsen, E., Mintenig, S.M., Besseling, E., and Koelmans, A.A., 2018, Quality criteria for the analysis of microplastic in biota samples—A critical review: Environmental Science & Technology, v. 52, no. 18, p. 10230–10240. [Also available at https://doi.org/10.1021/acs.est.8b01611.]
Hidalgo-Ruz, V., Gutow, L., Thompson, R.C., and Thiel, M., 2012, Microplastics in the marine environment—A review of the methods used for identification and quantification: Environmental Science & Technology, v. 46, no. 6, p. 3060–3075. [Also available at https://doi.org/10.1021/es2031505.]
Hong, S.H., Shim, W.J., and Hong, L., 2017, Methods of analysing chemicals associated with microplastics—A review: Analytical Methods, v. 9, no. 9, p. 1361–1368. [Also available at https://doi.org/10.1039/C6AY02971J.]
Horn, D.A., Granek, E.F., and Steele, C.L., 2020, Effects of environmentally relevant concentrations of microplastic fibers on Pacific mole crab (Emerita analoga) mortality and reproduction: Limnology and Oceanography Letters, v. 5, no. 1, p. 74–83. [Also available at https://doi.org/10.1002/lol2.10137.]
Horton, A.A., and Dixon, S.J., 2018, Microplastics—An introduction to environmental transport processes: WIREs, v. 5, no. 2, article e1268. [Also available at https://doi.org/10.1002/wat2.1268.]
Hu, X., Waigi, M.G., Yang, B., and Gao, Y., 2022, Impact of plastic particles on the horizontal transfer of antibiotic resistance genes to bacterium—Dependent on particle sizes and antibiotic resistance gene vector replication capacities: Environmental Science & Technology, v. 56, no. 21, p. 14948–14959. [Also available at https://doi.org/10.1021/acs.est.2c00745.]
Huang, Q., Lin, Y., Zhong, Q., Ma, F., and Zhang, Y., 2020, The impact of microplastic particles on population dynamics of predator and prey—Implication of the Lotka-Volterra model: Scientific Reports, v. 10, no. 1, p. 1–10. [Also available at https://doi.org/10.1038/s41598-020-61414-3.]
Huang, W., Song, B., Liang, J., Niu, Q., Zeng, G., Shen, M., Deng, J., Luo, Y., Wen, X., and Zhang, Y., 2021, Microplastics and associated contaminants in the aquatic environment—A review on their ecotoxicological effects, trophic transfer, and potential impacts to human health: Journal of Hazardous Materials, v. 405, article 124187. [Also available at https://doi.org/10.1016/j.jhazmat.2020.124187.]
Humphreys, J.M., Douglas, D.C., Ramey, A.M., Mullinax, J.M., Soos, C., Link, P., Walther, P., and Prosser, D.J., 2021, The spatial-temporal relationship of blue-winged teal to domestic poultry—Movement state modeling of a highly mobile avian influenza host: Journal of Applied Ecology, v. 58, no. 10, p. 2040–2052. [Also available at https://doi.org/10.1111/1365-2664.13963.]
Imran, M., Das, K.R., and Naik, M.M., 2019, Co-selection of multi-antibiotic resistance in bacterial pathogens in metal and microplastic contaminated environments—An emerging health threat: Chemosphere, v. 215, p. 846–857. [Also available at https://doi.org/10.1016/j.chemosphere.2018.10.114.]
Ivleva, N.P., Wiesheu, A.C., and Niessner, R., 2017, Microplastic in aquatic ecosystems: Angewandte Chemie, v. 56, no. 7, p. 1720–1739. [Also available at https://doi.org/10.1002/anie.201606957.]
Jabeen, K., Su, L., Li, J., Yang, D., Tong, C., Mu, J., and Shi, H., 2017, Microplastics and mesoplastics in fish from coastal and fresh waters of China: Environmental Pollution, v. 221, p. 141–149. [Also available at https://doi.org/10.1016/j.envpol.2016.11.055.]
Jang, M., Shim, W.J., Han, G.M., Rani, M., Song, Y.K., and Hong, S.H., 2016, Styrofoam debris as a source of hazardous additives for marine organisms: Environmental Science & Technology, v. 50, no. 10, p. 4951–4960. [Also available at https://doi.org/10.1021/acs.est.5b05485.]
Jenner, L.C., Rotchell, J.M., Bennett, R.T., Cowen, M., Tentzeris, V., and Sadofsky, L.R., 2022, Detection of microplastics in human lung tissue using μFTIR spectroscopy: Science of the Total Environment, v. 831, article 154907, accessed March 22, 2024, at https://doi.org/10.1016/j.scitotenv.2022.154907.]
Jeong, C.-B., Kang, H.-M., Lee, M.-C., Kim, D.-H., Han, J., Hwang, D.-S., Souissi, S., Lee, S.-J., Shin, K.-H., Park, H.G., and Lee, J.-S., 2017, Adverse effects of microplastics and oxidative stress-induced MAPK/Nrf2 pathway-mediated defense mechanisms in the marine copepod Paracyclopina nana: Nature, v. 7, no. 1, article 41323. [Also available at https://doi.org/10.1038/srep41323.]
Jin, T., Tang, J., Lyu, H., Wang, L., Gillmore, A.B., and Schaeffer, S.M., 2022, Activities of microplastics (MPs) in agricultural soil—A review of MPs pollution from the perspective of agricultural ecosystems: Journal of Agricultural and Food Chemistry, v. 70, no. 14, p. 4182–4201. [Also available at https://doi.org/10.1021/acs.jafc.1c07849.]
Kaile, N., Lindivat, M., Elio, J., Thuestad, G., Crowley, Q.G., and Hoell, I.A., 2020, Preliminary results from detection of microplastics in liquid samples using flow cytometry: Frontiers in Marine Science, v. 7. [Also available at https://doi.org/10.3389/fmars.2020.552688.]
Kapp, K.J., and Miller, R.Z., 2020, Electric clothes dryers—An underestimated source of microfiber pollution: PLoS ONE, v. 15, no. 10, article e0239165. [Also available at https://doi.org/10.1371/journal.pone.0239165.]
Käppler, A., Fischer, D., Oberbeckmann, S., Schernewski, G., Labrenz, M., Eichhorn, K.J., and Voit, B., 2016, Analysis of environmental microplastics by vibrational microspectroscopy—FTIR, Raman or both?: Analytical and Bioanalytical Chemistry, v. 408, no. 29, p. 8377–8391. [Also available at https://doi.org/10.1007/s00216-016-9956-3.]
Khaled, A., Rivaton, A., Richard, C., Jaber, F., and Sleiman, M., 2018, Phototransformation of plastic containing brominated flame retardants—Enhanced fragmentation and release of photoproducts to water and air: Environmental Science & Technology, v. 52, no. 19, p. 11123–11131. [Also available at https://doi.org/10.1021/acs.est.8b03172.]
Khoshmanesh, M., Sanati, A.M., and Ramavandi, B., 2023, Co-occurrence of microplastics and organic/inorganic contaminants in organisms living in aquatic ecosystems—A review: Marine Pollution Bulletin, v. 187, 114563. [Also available at https://doi.org/10.1016/j.marpolbul.2022.114563.]
Kirstein, I.V., Hensel, F., Gomiero, A., Iordachescu, L., Vianello, A., Wittgren, H.B., and Vollertsen, J., 2021, Drinking plastics?—Quantification and qualification of microplastics in drinking water distribution systems by µFTIR and Py-GCMS: Water Research, v. 188, article 116519. [Also available at https://doi.org/10.1016/j.watres.2020.116519.]
Kiryu, I., Ototake, M., Nakanishi, T., and Wakabayashi, H., 2000, The uptake of fluorescent microspheres into the skin, fins and gills of rainbow trout during immersion: Fish Pathology, v. 35, no. 1, p. 41–48. [Also available at https://doi.org/10.3147/jsfp.35.41.]
Koelmans, A.A., Bakir, A., Burton, G.A., and Janssen, C.R., 2016, Microplastic as a vector for chemicals in the aquatic environment—Critical review and model-supported reinterpretation of empirical studies: Environmental Science & Technology, v. 50, no. 7, p. 3315–3326. [Also available at https://doi.org/10.1021/acs.est.5b06069.]
Koelmans, A.A., Nor, N.H.M., Hermsen, E., Kooi, M., Mintenig, S.M., and France, J. de, 2019, Microplastics in freshwaters and drinking water—Critical review and assessment of data quality: Water Research, v. 155, p. 410–422. [Also available at https://doi.org/10.1016/j.watres.2019.02.054.]
Kolandhasamy, P., Su, L., Li, J., Qu, X., Jabeen, K., Shi, H., 2018, Adherence of microplastics to soft tissue of mussels—A novel way to uptake microplastics beyond ingestion: Science of the Total Environment, v. 610–611, p. 635–640. [Also available at https://doi.org/10.1016/j.scitotenv.2017.08.053.]
Kolomijeca, A., Parrott, J., Khan, H., Shires, K., Clarence, S., Sullivan, C., Chibwe, L., Sinton, D., and Rochman, C.M., 2020, Increased temperature and turbulence alter the effects of leachates from tire particles on fathead minnow (Pimephales promelas): Environmental Science & Technology, v. 54, no. 3, p. 1750–1759. [Also available at https://doi.org/10.1021/acs.est.9b05994.]
Krabbenhoft, T.J., Myers, B.J.E., Wong, J.P., Chu, C., Tingley, R.W., III, Falke, J.A., Kwak, T.J., Paukert, C.P., and Lynch, A.J., 2020, FiCli, the fish and climate change database, informs climate adaptation and management for freshwater fishes: Nature, v. 7, no. 1, article 124. [Also available at https://doi.org/10.1038/s41597-020-0465-z.]
Kraus, J.M., 2019. Contaminants in linked aquatic-terrestrial ecosystems—Predicting effects of aquatic pollution on adult aquatic insects and terrestrial insectivores: Freshwater Science, v. 38, no. 4, p. 919–927. [Also available at https://doi.org/10.1086/705997.]
Kraus, J.M., Walters, D.M., Wesner, J.S., Stricker, C.A., Schmidt, T.S., and Zuellig, R.E., 2014, Metamorphosis alters contaminants and chemical tracers in insects—Implications for food webs: Environmental Science & Technology, v. 48, no. 18, p. 10957–10965. [Also available at https://doi.org/10.1021/es502970b.]
Krause, S., Baranov, V., Nel, H.A., Drummond, J.D., Kukkola, A., Hoellein, T., Sambroke Smith, G.H., Lewandowski, J., Bonet, B., Packman, A.I., Sadler, J., Inshyna, V., Allen, S., Allen, D., Simon, L., Mermillod-Blondin, F., and Lynch, I., 2021, Gathering at the top? Environmental controls of microplastic uptake and biomagnification in freshwater food webs: Environmental Pollution, v. 268, pt. A, article 115750. [Also available at https://doi.org/10.1016/j.envpol.2020.115750.]
Kukkola, A., Runkel, R.L., Schneidewind, U., Murphy, S.F., Kelleher, L., Sambrook Smith, G.H., Nel, H.A., Lynch, I., and Krause, S., 2023, Prevailing impacts of river management on microplastic transport in contrasting U.S. streams—Rethinking global microplastic flux estimations: Water Research, v. 240, article 120112. [Also available at https://doi.org/10.1016/j.watres.2023.120112.]
Lambert, S., and Wagner, M., 2017, Microplastics are contaminants of emerging concern in freshwater environments—An overview, in Wagner, M., Lambert, S., eds., Freshwater Microplastics: The Handbook of Environmental Chemistry, v. 58, accessed March 22, 2024, at https://doi.org/10.1007/978-3-319-61615-5_1.
Lei, L., Wu, S., Shibo, L., Liu, M., Song, Y., Fu, Z., Shi, H., Raley-Susman, K.M., and He, D., 2018, Microplastic particles cause intestinal damage and other adverse effects in zebrafish Danio rerio and nematode Caenorhabditis elegans: Science of the Total Environment, v. 619–620, p. 1–8. [Also available at https://doi.org/10.1016/j.scitotenv.2017.11.103.]
Lenaker, P.L., Baldwin, A.K., Corsi, S.R., Mason, S.A., Reneau, P.C., and Scott, J.W., 2019, Vertical distribution of microplastics in the water column and surficial sediment from the Milwaukee River Basin to Lake Michigan: Environmental Science & Technology, v. 53, no. 21, p. 12227–12237. [Also available at https://doi.org/10.1021/acs.est.9b03850.]
Lenaker, P.L., Corsi, S.R., and Mason, S.A., 2021, Spatial distribution of microplastics in surficial benthic sediment of Lake Michigan and Lake Erie: Environmental Science & Technology, v. 55, no. 1, p. 373–384. [Also available at https://doi.org/10.1021/acs.est.0c06087.]
Leslie, H.A., Velzen, M.J.M. van, Brandsma, S.H., Vethaak, A.D., Garcia-Vallejo, J.J., and Lamoree, M.H., 2022, Discovery and quantification of plastic particle pollution in human blood: Environment International, v. 163, article 107199. [Also available at https://doi.org/10.1016/j.envint.2022.107199.]
Li, J., Green, C., Reynolds, A., Shi, H., and Rotchell, J.M., 2018, Microplastics in mussels sampled from coastal waters and supermarkets in the United Kingdom: Environmental Pollution, v. 241, p. 35–44. [Also available at https://doi.org/10.1016/j.envpol.2018.05.038.]
Liu, G., Zhu, Z., Yang, Y., Sun, Y., Yu, F., and Ma, J., 2019, Sorption behavior and mechanism of hydrophilic organic chemicals to virgin and aged microplastics in freshwater and seawater: Environmental Pollution, v. 246, p. 26–33. [Also available at https://doi.org/10.1016/j.envpol.2018.11.100.]
Liu, H., Yang, X., Liu, G., Liang, C., Xue, S., Chen, H., Ritsema, C.J., and Geissen, V., 2017, Response of soil dissolved organic matter to microplastic addition in Chinese loess soil: Chemosphere, v. 185, p. 907–917. [Also available at https://doi.org/10.1016/j.chemosphere.2017.07.064.]
Liu, W., Zhao, Y., Shi, Z., Li, Z., and Liang, X., 2020, Ecotoxicoproteomic assessment of microplastics and plastic additives in aquatic organisms—A review: Comparative Biochemistry and Physiology Part D: Genomics and Proteomics, v. 36, article 100713, accessed November 3, 2023, at https://doi.org/10.1016/j.cbd.2020.100713.
Löder, M.G.J., Imhof, H.K., Ladehoff, M., Löschel, L.A., Lorenz, C., Mintenig, S., Piehl, S., Primpke, S., Schrank, I., Laforsch, C., and Gerdts, G., 2017, Enzymatic purification of microplastics in environmental samples: Environmental Science & Technology, v. 51, no. 24, p. 14283–14292. [Also available at https://doi.org/10.1021/acs.est.7b03055.]
Long, M., Paul-Pont, I., Hégaret, H., Moriceau, B., Lambert, C., Huvet, A., and Soudant, P., 2017, Interactions between polystyrene microplastics and marine phytoplankton lead to species-specific hetero-aggregation: Environmental Pollution, v. 228, p. 454–463. [Also available at https://doi.org/10.1016/j.envpol.2017.05.047.]
Lu, L., Luo, T., Zhao, Y., Cai, C., Fu, Z., and Jin, Y., 2019, Interaction between microplastics and microorganism as well as gut microbiota—A consideration on environmental animal and human health: Science of the Total Environment, v. 667, p. 94–100. [Also available at https://doi.org/10.1016/j.scitotenv.2019.02.380.]
Lu, Y., Zhang, Y., Den, Y., Jiang, W., Zhao, Y., Geng, J., Ding, L., and Ren, H., 2016, Uptake and accumulation of polystyrene microplastics in zebrafish (Danio rerio) and toxic effects in liver: Environmental Science & Technology, v. 50, no. 7, p. 4054–4060. [Also available at https://doi.org/10.1021/acs.est.6b00183.]
Luo, H., Liu, C., He, D., Xu, J., Sun, J., Li, J., and Pan, X., 2022, Environmental behaviors of microplastics in aquatic systems—A systematic review on degradation, adsorption, toxicity and biofilm under aging conditions: Journal of Hazardous Materials, v. 423, pt. A, article 126915. [Also available at https://doi.org/10.1016/j.jhazmat.2021.126915.]
Lynch, J.M., 2019, Standards for measurement science of microplastic pollution, in The Science of Microplastics in the World Ocean—Program [An International Workshop To Formulate Next Steps in Understanding the Fate, Distribution, Impacts, and Technology Development Necessary To Push the Science], Woods Hole, Massachusetts, October 15, 2019: Woods Hole, Mass., Woods Hole Oceanographic Institution, 45-slide presentation, accessed October 15, 2019, at https://microplastics.whoi.edu/the-science-of-microplastics-in-the-world-ocean-international-workshop/program/.
Ma, H., Pu, S., Liu, S., Bai, Y., Mandal, S., and Xing, B., 2020, Microplastics in aquatic environments—Toxicity to trigger ecological consequences: Environmental Pollution, v. 261, 114089. [Also available at https://doi.org/10.1016/j.envpol.2020.114089.
Maes, T., Jessop, R., Wellner, N., Haupt, K., and Mayes, A.G., 2017, A rapid-screening approach to detect and quantify microplastics based on fluorescent tagging with Nile Red: Nature, v. 7, no. 1, article 44501. [Also available at https://doi.org/10.1038/srep44501.
Mahon, A.M., O’Connell, B., Healy, M.G., O’Connor, I., Officer, R., Nash, R., and Morrison, L., 2017, Microplastics in sewage sludge—Effects of treatment: Environmental Science & Technology, v. 51, no. 2, p. 810–818. [Also available at https://doi.org/10.1021/acs.est.6b04048.
Marsden, P., Koelmans, B., Bourdon-Lacombe, J., Gouin, T., D’Anglada, L., Cunliffe, D., Jarvis, P., Fawell, J., and France, J. de, 2019, Microplastics in drinking water: Geneva, Switzerland, World Health Organization, 124 p. [Also available at https://iris.who.int/bitstream/handle/10665/326499/9789241516198-eng.pdf?sequence=5.]
Martins, A., and Guilhermino, L., 2018, Transgenerational effects and recovery of microplastics exposure in model populations of the freshwater cladoceran Daphnia magna Straus: Science of the Total Environment, v. 631–632, p. 421–428. [Also available at https://doi.org/10.1016/j.scitotenv.2018.03.054.]
Mason, S.A., Garneau, D., Sutton, R., Chu, Y., Ehmann, K., Barnes, J., Fink, P., Papazissimos, D., and Rogers, D.L., 2016, Microplastic pollution is widely detected in U.S. municipal wastewater treatment plant effluent: Environmental Pollution, v. 218, p. 1045–1054. [Also available at https://doi.org/10.1016/j.envpol.2016.08.056.]
Masoner, J.R., Kolpin, D.W., Cozzarelli, I.M., Smalling, K.L., Bolyard, S.C., Field, J.A., Furlong, E.T., Gray, J.L., Lozinski, D., Reinhart, D., Rodowa, A., and Bradley, P.M., 2020, Landfill leachate contributes per-/poly-fluoroalkyl substances (PFAS) and pharmaceuticals to municipal wastewater: Environmental Science, v. 6, no. 5, p. 1300–1311. [Also available at https://doi.org/10.1039/D0EW00045K.]
Masry, M., Rossignol, S., Gardette, J.-L., Therias, S., Bussière, P.-O., and Wong-Wah-Chung, P., 2021, Characteristics, fate, and impact of marine plastic debris exposed to sunlight—A review: Marine Pollution Bulletin, v. 171, article 112701. [Also available at https://doi.org/10.1016/j.marpolbul.2021.112701.]
Masura, J., Baker, J., Foster, G., Arthur, C., 2015, Laboratory methods for the analysis of microplastics in the marine environment—Recommendations for quantifying synthetic particles in waters and sediments: NOAA [National Oceanic and Atmospheric Administration] Technical Memorandum NOS–OR&R–48, 31 p. [Also available at https://repository.oceanbestpractices.org/handle/11329/1076.]
McCormick, A.R., Hoellein, T.J., London, M.G., Hittie, J., Scott, J.W., and Kelly, J.J., 2016, Microplastic in surface waters of urban rivers—Concentrations, sources, and associated bacterial assemblages: Ecosphere, v. 7, no. 11, article e01556. [Also available at https://doi.org/10.1002/ecs2.1556.]
McDuie, F., Matchett, E.L., Prosser, D.L., Takekawa, J.Y., Pitesky, M.E., Lorenz, A.A., McCuen, M.M., Overton, C.T., Ackerman, J.T., La Cruz, S.E.W. de, and Casazza, M.L., 2022, Pathways for avian influenza virus spread—GPS reveals wild waterfowl in commercial livestock facilities and connectivity with the natural wetland landscape: Transboundary and Emerging Diseases, v. 69, no. 5, p. 2898–2912. [Also available at https://doi.org/10.1111/tbed.14445.]
McIlwraith, H.K., Kim, J., Helm, P., Bhavsar, S.P., Metzger, J.S., and Rochman, C.M., 2021, Evidence of microplastic translocation in wild-caught fish and implications for microplastic accumulation dynamics in food webs: Environmental Science & Technology, v. 55, no. 18, p. 12372–12382. [Also available at https://doi.org/10.1021/acs.est.1c02922.]
McIntyre, J.K., Prat, J., Cameron, J., Wetzel, J., Mudrock, E., Peter, K.T., Tian, Z., Mackenzie, C., Lundin, J., Stark, J.D., King, K., Davis, J.W., Kolodziej, E.P., and Scholz, N.L., 2021, Treading water—Tire wear particle leachate recreates an urban runoff mortality syndrome in Coho but not chum salmon: Environmental Science & Technology, v. 55, no. 17, p. 11767–11774. [Also available at https://doi.org/10.1021/acs.est.1c03569.]
McNeish, R.E., Kim, L.H., Barrett, H.A., Mason, S.A., Kelly, J.J., and Hoellein, T.J., 2018, Microplastic in riverine fish is connected to species traits: Nature, v. 8, no. 1, article 11639. [Also available at https://doi.org/10.1038/s41598-018-29980-9.]
Miller, M.E., Hamann, M., and Kroon, F.J., 2020, Bioaccumulation and biomagnification of microplastics in marine organisms—A review and meta-analysis of current data: PLoS ONE, v. 15, no. 10, article e0240792. [Also available at https://doi.org/10.1371/journal.pone.0240792.]
Minnesota Department of Health, 2019, Microplastics and nanoplastics in the environment: Minnesota Department of Health website, accessed January 5, 2024, at https://www.house.mn.gov/comm/docs/67f7ca46-d89f-42a1-9b2a-7bb777d32d2f.pdf.
Mizraji, R., Ahrendt, C., Perez-Venegas, D., Vargas, J., Pulgar, J., Aldana, M., Ojeda, F.P., Duarte, C., and Galbán-Malagón, C., 2017, Is the feeding type related with the content of microplastics in intertidal fish gut?: Marine Pollution Bulletin, v. 116, no. 1–2, p. 498–500. [Also available at https://doi.org/10.1016/j.marpolbul.2017.01.008.]
Mueller, M.-T., Fueser, H., Höss, S., and Traunspurger, W., 2020, Species-specific effects of long-term microplastic exposure on the population growth of nematodes, with a focus on microplastic ingestion: Ecological Indicators, v. 118, article 106698. [Also available at https://doi.org/10.1016/j.ecolind.2020.106698.]
National Atmospheric Deposition Program, 2022, Operator support, national trends network: National Atmospheric Deposition Program website, accessed May 3, 2022, at https://nadp.slh.wisc.edu/siteops/.
National Nanotechnology Initiative, 2024, Federal communities of interest: National Nanotechnology Initiative website, accessed January 4, 2024, at https://www.nano.gov/federalcommunitiesofinterest.
Nizzetto, L., Futter, M., and Langaas, S., 2016, Are agricultural soils dumps for microplastics of urban origin?: Environmental Science & Technology, v. 50, no. 20, p. 10777–10779. [Also available at https://doi.org/10.1021/acs.est.6b04140.]
Oberbeckmann, S., Kreikemeyer, B., and Labrenz, M., 2018, Environmental factors support the formation of specific bacterial assemblages on microplastics: Frontiers in Microbiology, v. 8, article 2709, 12 p. [Also available at https://doi.org/10.3389/fmicb.2017.02709.]
Ogonowski, M., Gerdes, Z., and Gorokhova, E., 2018, What we know and what we think we know about microplastic effects—A critical perspective: Current Opinion in Environmental Science & Health, v. 1, p. 41–46. [Also available at https://doi.org/10.1016/j.coesh.2017.09.001.]
Okoffo, E.D., Tscharke, B.J., O’Brien, J.W., O’Brien, S., Ribeiro, F., Burrows, S.D., Choi, P.M., Wang, X., Mueller, J.F., and Thomas, K.V., 2020, Release of plastics to Australian land from biosolids end-use: Environmental Science & Technology, v. 54, no. 23, p. 15132–15141, accessed at https://doi.org/10.1021/acs.est.0c05867.
Oliveira, M., Ameixa, O.M.C.C., and Soares, A.M.V.M., 2019, Are ecosystem services provided by insects “bugged” by micro (nano) plastics?: Trends in Analytical Chemistry, v. 113, p. 317–320. [Also available at https://doi.org/10.1016/j.trac.2019.02.018.]
Organisation for Economic Co-operation and Development, 2019, Improving resource efficiency to combat marine plastic litter: Paris, France, Organisation for Economic Co-operation and Development, 25 p. [Also available at https://www.oecd.org/g20/summits/osaka/OECD-G20-Paper-Resource-Efficiency-and-Marine-Plastics.pdf.]
Paluselli, A., Fauvelle, V., Galgani, F., and Sempéré, R., 2019, Phthalate release from plastic fragments and degradation in seawater: Environmental Science & Technology, v. 53, no. 1, p. 166–175, accessed March 22, 2024, at https://doi.org/10.1021/acs.est.8b05083.
Panko, J.M., Kreider, M.L., McAtee, B.L., and Marwood, C., 2013, Chronic toxicity of tire and road wear particles to water- and sediment-dwelling organisms (corrected May 16, 2020): Ecotoxicology, v. 22, no. 1, p. 13–21. [Also available at https://doi.org/10.1007/s10646-012-0998-9.]
Panno, S.V., Kelly, W.R., Scott, J., Zheng, W., McNeish, R.E., Holm, N., Hoellein, T.J., and Baranski, E.L., 2019, Microplastic contamination in karst groundwater systems: Groundwater, v. 57, no. 2, p. 189–196. [Also available at https://doi.org/10.1111/gwat.12862.]
Peiponen, K.-E., Asamoah, B.O., Kanyathare, B., Räty, J., and Roussey, M., 2020, Detecting microplastics with optics: Optics and Photonics News, v. 31, no. 11, p. 24–31. [Also available at https://doi.org/10.1364/OPN.31.11.000024.]
Peng, G., Bellerby, R., Zhang, F., Sun, X., and Li, D., 2020, The ocean’s ultimate trashcan—Hadal trenches as major depositories for plastic pollution: Water Research, v. 168, article 115121. [Also available at https://doi.org/10.1016/j.watres.2019.115121.]
Pham, D.N., Clark, L., and Li, M., 2021, Microplastics as hubs enriching antibiotic-resistant bacteria and pathogens in municipal activated sludge: Journal of Hazardous Materials Letters, v. 2, article 100014. [Also available at https://doi.org/10.1016/j.hazl.2021.100014.]
Prata, J.C., Costa, J.P. da, Duarte, A.C., and Rocha-Santos, T., 2019a, Methods for sampling and detection of microplastics in water and sediment—A critical review: Trends in Analytical Chemistry, v. 110, p. 150–159. [Also available at https://doi.org/10.1016/j.trac.2018.10.029.]
Prata, J.C., Costa, J.P. da, Lopes, I., Duarte, A.C., and Rocha-Santos, T., 2019b, Effects of microplastics on microalgae populations—A critical review: Science of the Total Environment, v. 665, p. 400–405. [Also available at https://doi.org/10.1016/j.scitotenv.2019.02.132.]
Prata, J.C., Costa, J.P. da, Lopes, I., Duarte, A.C., and Rocha-Santos, T., 2020, Environmental exposure to microplastics—An overview on possible human health effects: Science of the Total Environment, v. 702, article 134455. [Also available at https://doi.org/10.1016/j.scitotenv.2019.134455.]
Primpke, S., Christiansen, S.H., Cowger, W., Frond, H. de, Deshpande, A., Fischer, M., Holland, E.B., Meyns, M., O’Donnell, B.A., Ossmann, B.E., Pttroff, M., Sarau, G., Scholz-Böttcher, B.M., and Wiggin, K.J., 2020, Critical assessment of analytical methods for the harmonized and cost-efficient analysis of microplastics: Applied Spectroscopy, v. 74, no. 9, p. 1012–1047. [Also available at https://doi.org/10.1177/0003702820921465.]
Primpke, S., Lorenz, C., Rascher-Friesenhausen, R., and Gerdts, G., 2017, An automated approach for microplastics analysis using focal plane array (FPA) FTIR microscopy and image analysis: Analytical Methods, v. 9, no. 9, p. 1499–1511. [Also available at https://doi.org/10.1039/C6AY02476A.]
Prokić, M.D., Radovanović, T.B., Gavrić, J.P., and Faggio, C., 2019, Ecotoxicological effects of microplastics—Examination of biomarkers, current state and future perspectives: Trends in Analytical Chemistry, v. 111, p. 37–46. [Also available at https://doi.org/10.1016/j.trac.2018.12.001.]
Provencher, J.F., Ammendolia, J., Rochman, C.M., and Mallory, M.L., 2019, Assessing plastic debris in aquatic food webs—What we know and don’t know about uptake and trophic transfer: Environmental Reviews, v. 27, no. 3, p. 304–317. [Also available at https://doi.org/10.1139/er-2018-0079.
Pulster, E.L., Rullo, K., Gilbert, S., Ash, T.M., Goetting, B., Campbell, K., Markham, S., and Murawski, S.A., 2022, Assessing per- and polyfluoroalkyl substances (PFAS) in sediments and fishes in a large, urbanized estuary and the potential human health implications: Frontiers in Marine Science, v. 9, article 1046667. [Also available at https://doi.org/10.3389/fmars.2022.1046667.]
Qi, R., Jones, D.L., Li, Z., Liu, Q., and Yan, C., 2020, Behavior of microplastics and plastic film residues in the soil environment—A critical review: Science of the Total Environment, v. 703, article 134722. [Also available at https://doi.org/10.1016/j.scitotenv.2019.134722.]
Qiao, R., Sheng, C., Lu, Y., Zhang, Y., Ren, H., and Lemos, B., 2019, Microplastics induce intestinal inflammation, oxidative stress, and disorders of metabolome and microbiome in zebrafish: Science of the Total Environment, v. 662, p. 246–253. [Also available at https://doi.org/10.1016/j.scitotenv.2019.01.245.]
Ragusa, A., Svelato, A., Santacroce, C., Catalano, P., Notarstefano, V., Carnevali, O., Papa, F., Rongioletti, M.C.A., Baiocco, F., Draghi, S., D’Amore, E., Rinaldo, D., Matta, M., and Giorgini, E., 2021, Plasticenta—First evidence of microplastics in human placenta: Environment International, v. 146, article 106274. [Also available at https://doi.org/10.1016/j.envint.2020.106274.]
Ramey, A.M., Hill, N.J., DeLiberto, T.J., Gibbs, S.E.J., Hopkins, C.M., Lang, A.S., Poulson, R.L., Prosser, D.J., Sleeman, J.M., Stallknecht, D.E., and Wan, X.-F., 2022, Highly pathogenic avian influenza is an emerging disease threat to wild birds in North America: The Journal of Wildlife Management, v. 86, no. 2, article e22171. [Also available at https://doi.org/10.1002/jwmg.22171.]
Redondo-Hasselerharm, P.E., de Ruijter, V.N., Mintenig, S.M., Verschoor, A., and Koelmans, A.A., 2018, Ingestion and chronic effects of car tire tread particles on freshwater benthic macroinvertebrates: Environmental Science & Technology, v. 52, no. 23, p. 13986–13994. [Also available at https://doi.org/10.1021/acs.est.8b05035.]
Redondo-Hasselerharm, P.E., Gort, G., Peeters, E.T.H.M., and Koelmans, A.A., 2020, Nano- and microplastics affect the composition of freshwater benthic communities in the long term: Science Advances, v. 6, no. 5, article eaay4054. [Also available at https://doi.org/10.1126/sciadv.aay4054.]
Rehse, S., Kloas, W., and Zarfl, C., 2018, Microplastics reduce short-term effects of environmental contaminants. Part 1—Effects of bisphenol A on freshwater zooplankton are lower in presence of polyamide particles: International Journal of Environmental Research and Public Health, v. 15, no. 2, article 280. [Also available at https://doi.org/10.3390/ijerph15020280.]
Rezaei, M., Abbasi, S., Pourmahmood, H., Oleszczuk, P., Ritsema, C., and Turner, A., 2022, Microplastics in agricultural soils from a semi-arid region and their transport by wind erosion: Environmental Research, v. 212, pt. B, article 113213. [Also available at https://doi.org/10.1016/j.envres.2022.113213.]
Roch, S., Friedrich, C., and Brinker, A., 2020, Uptake routes of microplastics in fishes—Practical and theoretical approaches to test existing theories: Nature, v. 10, no. 1, article 3896. [Also available at https://doi.org/10.1038/s41598-020-60630-1.]
Rocha-Santos, T., and Duarte, A.C., 2015, A critical overview of the analytical approaches to the occurrence, the fate and the behavior of microplastics in the environment: Trends in Analytical Chemistry, v. 65, p. 47–53. [Also available at https://doi.org/10.1016/j.trac.2014.10.011.]
Rochman, C.M., Brookson, C., Bikker, J., Djuric, N., Earn, A., Bucci, K., Athey, S., Huntington, A., McIlwraith, H., Munno, K., Frond, H. de, Kolomijeca, A., Erdle, L., Grbic, J., Bayoumi, M., Borrelle, S.B., Wu, T., Santoro, S., Werbowski, L.M., Zhu, X., Giles, R.K., Hamilton, B.M., Thaysen, C., Kaura, A., Klasios, N., Ead, L., Kim, J., Sherlock, C., Ho, A., and Hung, C., 2019, Rethinking microplastics as a diverse contaminant suite: Environmental Toxicology and Chemistry, v. 38, no. 4, p. 703–711. [Also available at https://doi.org/10.1002/etc.4371.]
Rochman, C.M., Hentschel, B.T., and Teh, S.J., 2014, Long-term sorption of metals is similar among plastic types—Implications for plastic debris in aquatic environments: PLoS ONE, v. 9, no. 1, article e85433. [Also available at https://doi.org/10.1371/journal.pone.0085433.]
Rochman, C.M., Kross, S.M., Armstrong, J.B., Bogan, M.T., Darling, E.S., Green, S.J., Smyth, A.R., and Veríssimo, D., 2015, Scientific evidence supports a ban on microbeads: Environmental Science & Technology, v. 49, no. 18, p. 10759–10761. [Also available at https://doi.org/10.1021/acs.est.5b03909.]
Roman, L., Gilardi, K., Lowenstine, L., Hardesty, B.D., and Wilcox, C., 2021, The need for attention to conformation bias and confounding in the field of plastic pollution and wildlife impacts—Comment on “clinical pathology of plastic ingestion in marine birds and relationships with blood chemistry”: Environmental Science & Technology. [Also available at https://doi.org/10.1021/acs.est.0c02874.]
Roy, P.K., Hakkarainen, M., Varma, I.K., and Albertsson, A.-C., 2011, Degradable polyethylene—Fantasy or reality: Environmental Science & Technology, v. 45, no. 10, p. 4217–4227. [Also available at https://doi.org/10.1021/es104042f.]
Ryan, P.G., 2015, A brief history of marine litter research, chap. 1 of Bergmann, M., Gutow, L., and Klages, M., eds., Marine anthropogenic litter: Cham, Switzerland, Springer, p. 1–25. [Also available at https://doi.org/10.1007/978-3-319-16510-3_1.]
Ryan, P.G., and Moloney, C.L., 1990, Plastic and other artefacts on South African beaches—Temporal trends in abundance and composition: South African Journal of Science, v. 86, p. 450–452. [Also available at https://www.researchgate.net/publication/283507743_plastic_and_other_artefacts_on_South_African_beaches_temporal_trends_in_abundance_and_composition.]
Sarijan, S., Azman, S., Said, M.I.M., and Jamal, M.H., 2021, Microplastics in freshwater ecosystems—A recent review of occurrence, analysis, potential impacts, and research needs: Environmental Science and Pollution Research, v. 28, no. 2, p. 1341–1356. [Also available at https://doi.org/10.1007/s11356-020-11171-7.]
Schrank, I., Trotter, B., Dummert, J., Scholz-Böttcher, B.M., Löder, M.G.J., and Laforsch, C., 2019, Effects of microplastic particles and leaching additive on the life history and morphology of Daphnia magna: Environmental Pollution, v. 255, pt. 2, article 113233. [Also available at https://doi.org/10.1016/j.envpol.2019.113233.]
Scopetani, C., Esterhuizen-Londt, M., Chelazzi, D., Cincinelli, A., Setälä, H., and Pflugmacher, S., 2020, Self-contamination from clothing in microplastics research: Ecotoxicology and Environmental Safety, v. 189, article 110036. [Also available at https://doi.org/10.1016/j.ecoenv.2019.110036.]
Semcesen, P.O., and Wells, M.G., 2021, Biofilm growth on buoyant microplastics leads to changes in settling rates—Implications for microplastic retention in the Great Lakes: Marine Pollution Bulletin, v. 170, article 112573. [Also available at https://doi.org/10.1016/j.marpolbul.2021.112573.]
Seuront, L., 2018, Microplastic leachates impair behavioural vigilance and predator avoidance in a temperate intertidal gastropod: Biology Letters, v. 14, no. 11, article 20180453. [Also available at https://doi.org/10.1098/rsbl.2018.0453.]
Sgier, L., Freimann, R., Zupanic, A., and Kroll, A., 2016, Flow cytometry combined with viSNE for the analysis of microbial biofilms and detection of microplastics: Nature Communications, v. 7, no. 1, article 11587. [Also available at https://doi.org/10.1038/ncomms11587.]
Shim, W.J., Hong, S.H., and Eo, S.E., 2017, Identification methods in microplastic analysis—A review: Analytical Methods, v. 9, no. 9, p. 1384–1391. [Also available at https://doi.org/10.1039/C6AY02558G.]
Shim, W.J., Song, Y.K., Hong, S.H., and Jang, M., 2016, Identification and quantification of microplastics using Nile Red staining: Marine Pollution Bulletin, v. 113, no. 1–2, p. 469–476. [Also available at https://doi.org/10.1016/j.marpolbul.2016.10.049.]
Shimizu, K., Sokolov, S.V., Kätelhön, E., Holter, J., Young, N.P., and Compton, R.G., 2017, In situ detection of microplastics—Single microparticle‐electrode impacts: Electroanalysis, v. 29, no. 10, p. 2200–2207. [Also available at https://doi.org/10.1002/elan.201700213.]
Silva, A.B., Bastos, A.S., Justino, C.I.L., Costa, J.P. da, Duarte, A.C., and Rocha-Santos, T.A.P., 2018a, Microplastics in the environment—Challenges in analytical chemistry—A review: Analytica Chimica Acta, v. 1017, p. 1–19. [Also available at https://doi.org/10.1016/j.aca.2018.02.043.]
Silva, J.D.B., Barletta, M., Lima, A.R.A., and Ferreira, G.V.B., 2018b, Use of resources and microplastic contamination throughout the life cycle of grunts (Haemulidae) in a tropical estuary: Environmental Pollution, v. 242, pt. A, p. 1010–1021. [Also available at https://doi.org/10.1016/j.envpol.2018.07.038.]
Smith, E.C., and Turner, A., 2020, Mobilisation kinetics of Br, Cd, Cr, Hg, Pb and Sb in microplastics exposed to simulated, dietary-adapted digestive conditions of seabirds: Science of the Total Environment, v. 733, article 138802. [Also available at https://doi.org/10.1016/j.scitotenv.2020.138802.]
Smith, M., Love, D.C., Rochman, C.M., and Neff, R.A., 2018, Microplastics in seafood and the implications for human health: Current Environmental Health Reports, v. 5, no. 3, p. 375–386. [Also available at https://doi.org/10.1007/s40572-018-0206-z.]
Smyth, K., Drake, J., Li, Y., Rochman, C., Seters, T. van, and Passeport, E., 2021, Bioretention cells remove microplastics from urban stormwater: Water Research, v. 191, article 116785. [Also available at https://doi.org/10.1016/j.watres.2020.116785.]
Soares, M.D.O., Matos, E., Lucas, C., Rizzo, L., Allcock, L., and Rossi, S., 2020, Microplastics in corals—An emergent threat: Marine Pollution Bulletin, v. 161, pt. A, article 111810. [Also available at https://doi.org/10.1016/j.marpolbul.2020.111810.]
Sorensen, R.M., Kanwar, R.S., and Jovanovi, B., 2023, Past, present, and possible future policies on plastic use in the United States, particularly microplastics and nanoplastics—A review: Integrated Environmental Assessment and Management, v. 19, no. 2, p. 474–488. [Also available at https://doi.org/10.1002/ieam.4678.]
Spanjer, A.R., Liedtke, T.L., Conn, K.E., Weiland, L.K., Black, R.W., and Godfrey, N., 2020, Evidence for rapid gut clearance of microplastic polyester fibers fed to Chinook salmon—A tank study: Environmental Pollution, v. 265, pt. A, article 115083. [Also available at https://doi.org/10.1016/j.envpol.2020.115083.]
State of California, 2017a, SB–126 Budget act of 2017: Sacramento, Calif., California Legislature. [Also available at https://leginfo.legislature.ca.gov/faces/billTextClient.xhtml?bill_id=201720180SB126.]
State of California, 2017b, SB–1422 California safe drinking water act—Microplastics: Sacramento, Calif., California Legislature. [Also available at https://leginfo.legislature.ca.gov/faces/billTextClient.xhtml?bill_id=201720180SB1422.]
Stets, E.G., Sprague, L.A., Oelsner, G.P., Johnson, H.M., Murphy, J.C., Ryberg, K., Vecchia, A.V., Zuellig, R.E., Falcone, J.A., and Riskin, M.L., 2020, Landscape drivers of dynamic change in water quality of U.S. rivers: Environmental Science & Technology, v. 54, no. 7, p. 4336–4343. [Also available at https://doi.org/10.1021/acs.est.9b05344.]
Summers, S., Henry, T., and Gutierrez, T., 2018, Agglomeration of nano-and microplastic particles in seawater by autochthonous and de novo-produced sources of exopolymeric substances: Marine Pollution Bulletin, v. 130, p. 258–267. [Also available at https://doi.org/10.1016/j.marpolbul.2018.03.039.]
Susanti, N.K.Y., Mardiastuti, A., and Wardiatno, Y., 2020, Microplastics and the impact of plastic on wildlife—A literature review: IOP Conference Series—Earth and Environmental Science, v. 528, no. 1, article 012013. [Also available at https://doi.org/10.1088/1755-1315/528/1/012013.]
Sutton, R., Lin, D., Sedlak, M., Box, C., Gilbreath, A., Holleman, R., Miller, E., Wong, A., Munno, K., Zhu, X., Franz, A., and Rochman, C., 2019, Understanding microplastic levels, pathways, and transport in the San Francisco Bay region: Richmond, Calif., San Francisco Estuary Institute, 389 p. [Also available at https://www.sfei.org/sites/default/files/biblio_files/Microplastic%20Levels%20in%20SF%20Bay%20-%20Final%20Report.pdf.]
Tagg, A.S., Brandes, E., Fischer, F., Fischer, D., Brandt, J., and Labrenz, M., 2022, Agricultural application of microplastic-rich sea sludge led to further uncontrolled contamination: Science of the Total Environment, v. 806, pt. 4, article 150611. [Also available at https://doi.org/10.1016/j.scitotenv.2021.150611.]
Talsness, C.E., Andrade, A.J., Kuriyama, S.N., Taylor, J.A., and Vom Saal, F.S., 2009, Components of plastic—Experimental studies in animals and relevance for human health: Philosophical Transactions of the Royal Society of London B—Biological Sciences, v. 364, no. 1526, p. 2079–2096. [Also available at https://doi.org/10.1098/rstb.2008.0281.]
Talvitie, J., Mikola, A., Setälä, O., Heinonen, M., and Koistinen, A., 2017, How well is microlitter purified from wastewater?—A detailed study on the stepwise removal of microlitter in a tertiary level wastewater treatment plant: Water Research, v. 109, p. 164–172. [Also available at https://doi.org/10.1016/j.watres.2016.11.046.]
Tao, D., Zhang, K., Xu, S., Lin, H., Liu, Y., Kang, J., Yim, T., Giesy, J.P., and Leung, K.M.Y., 2022, Microfibers released into the air from a household tumble dryer: Environmental Science & Technology Letters, v. 9, no. 2, p. 120–126. [Also available at https://doi.org/10.1021/acs.estlett.1c00911.]
Terando, A., Reidmiller, D., Hostetler, S.W., Littell, J.S., Beard, D., Jr., Weiskopf, S.R., Belnap, J., and Plumlee, G.S., 2020, Using information from global climate models to inform policymaking—The role of the U.S. Geological Survey: U.S. Geological Survey Open-File Report 2020–1058, 32 p. [Also available at https://doi.org/10.3133/ofr20201058.]
Thornton Hampton, L.M., Lowman, H., Coffin, S., Darin, E., Frond, H. de, Hermabessiere, L., Miller, E., de Ruijter, V.N., Faltynkova, A., Kotar, S., Monclús, L., Siddiqui, S., Völker, J., Brander, S., Koelmans, A.A., Rochman, C.M., Wagner, M., and Mehinto, A.C., 2022, A living tool [Toxicity of Microplastics Explorer (ToMEx)] for the continued exploration of microplastic toxicity (corrected August 31, 2022): Microplastics and Nanoplastics, v. 2, article 13. [Also available at https://doi.org/10.1186/s43591-022-00032-4.]
Tian, Z., Zhao, H., Peter, K.T., Gonzalez, M., Wetzel, J., Wu, C., Hu, W., Prat, J., Mudrock, E., Hettinger, R., Cortina, A.E., Biswas, R.G., Kock, F.V.C., Soong, R., Jenne, A., Du, B., Hou, F., He, H., Lundeen, R., Gilbreath, A., Sutton, R., Scholz, N.L., Davis, J.W., Dodd, M.C., Simpson, A., McIntyre, J.K., and Kolodziej, E.P., 2021, A ubiquitous tire rubber-derived chemical induces acute mortality in coho salmon (corrected February 18, 2022): Science, v. 371, no. 6525, p. 185–189. [Also available at https://doi.org/10.1126/science.abd6951.]
Tokranov, A.K., Bradley, P.M., Focazio, M.J., Kent, D.B., LeBlanc, D.R., Smalling, K.R., Steevens, J.A., Toccalino, P.L., 2021, Integrated science for the study of perfluoroalkyl and polyfluoroalkyl substances (PFAS) in the environment—A strategic vision for the U.S. Geological Survey: U.S. Geological Survey Circular 1490, 50 p., accessed February 2, 2024, at https://doi.org/10.3133/cir1490.
Tourinho, P.S., Kočí, V., Loureiro, S., and van Gestel, C.A., 2019, Partitioning of chemical contaminants to microplastics—Sorption mechanisms, environmental distribution and effects on toxicity and bioaccumulation: Environmental Pollution, v. 252, pt. B, p. 1246–1256. [Also available at https://doi.org/10.1016/j.envpol.2019.06.030.]
Troost, T.A., Desclaux, T., Leslie, H.A., Meulen, M.D. van der, and Vethaak, A.D., 2018, Do microplastics affect marine ecosystem productivity?: Marine Pollution Bulletin, v. 135, p. 17–29. [Also available at https://doi.org/10.1016/j.marpolbul.2018.05.067.]
Turner, A., 2017, In situ elemental characterisation of marine microplastics by portable XRF: Marine Pollution Bulletin, v. 124, no. 1, p. 286–291. [Also available at https://doi.org/10.1016/j.marpolbul.2017.07.045.]
Turner, A., and Holmes, L.A., 2015, Adsorption of trace metals by microplastic pellets in fresh water: Environmental Chemistry, v. 12, no. 5, p. 600–610. [Also available at https://doi.org/10.1071/EN14143.]
Turner, A., Holmes, L., Thompson, R.C., and Fisher, A.S., 2020, Metals and marine microplastics—Adsorption from the environment versus addition during manufacture, exemplified with lead: Water Research, v. 173, article 115577. [Also available at https://doi.org/10.1016/j.watres.2020.115577.]
Turner, S., Horton, A.A., Rose, N.L., and Hall, C., 2019, A temporal sediment record of microplastics in an urban lake, London, UK: Journal of Paleolimnology, v. 61, no. 4, p. 449–462. [Also available at https://doi.org/10.1007/s10933-019-00071-7.]
U.S. Environmental Protection Agency, 2016, Basic information about biosolids: U.S. Environmental Protection Agency web page, accessed May 18, 2022, at https://www.epa.gov/biosolids/basic-information-about-biosolids.
U.S. Environmental Protection Agency, 2017, Microplastics expert workshop report: U.S. Environmental Protection Agency [Document], 37 p., accessed May 4, 2022, at https://www.epa.gov/sites/default/files/2018-03/documents/microplastics_expert_workshop_report_final_12-4-17.pdf.
U.S. Food and Drug Administration, 2023, The Microbead-Free Waters Act—FAQs: U.S. Food and Drug Administration web page, accessed November 3, 2023, at https://www.fda.gov/cosmetics/cosmetics-laws-regulations/microbead-free-waters-act-faqs.
U.S. Geological Survey, 2006, Collection of water samples (ver. 2.0, September 2006): U.S. Geological Survey Techniques of Water-Resources Investigations, book 9, chap. A4, accessed June 10, 2014, at https://doi.org/10.3133/twri09A4.
U.S. Geological Survey, 2022, Quality Management System for U.S. Geological Survey laboratories: U.S. Geological Survey Instructional Memorandum IM OSQI 2022–01, accessed January 4, 2024, at www.usgs.gov/survey-manual/im-osqi-2022-01.
Usman, S., Abdull Razis, A.F., Shaari, K., Azmai, M.N.A., Saad, M.Z., Mat Isa, N., and Nazarudin, M.F., 2022, The burden of microplastics pollution and contending policies and regulations: International Journal of Environmental Research and Public Health, v. 19, no. 11, article 6773. [Also available at https://pubmed.ncbi.nlm.nih.gov/35682361/.]
Van Colen, C., Vanhove, B., Diem, A., and Moens, T., 2020, Does microplastic ingestion by zooplankton affect predator-prey interactions? An experimental study on larviphagy: Environmental Pollution, v. 256, article 113479. [Also available at https://doi.org/10.1016/j.envpol.2019.113479.]
Walters, D.M., Otter, R.R., Kraus, J.M., and Mills, M.A., 2018, Riparian spiders indicate the magnitude and sources of polychlorinated biphenyl contamination at a large contaminated sediment site: Environmental Toxicology and Chemistry, v. 37, no. 9, p. 2467–2474. [Also available at https://doi.org/10.1002/etc.4216.]
Wang, J., Guo, X., and Xue, J., 2021a, Biofilm-developed microplastics as vectors of pollutants in aquatic environments: Environmental Science & Technology, v. 55, no. 19, p. 12780-12790, accessed September 28, 2021, at https://doi.org/10.1021/acs.est.1c04466.
Wang, K., Li, J., Zhao, L., Mu, X., Wang, C., Wang, M., Xue, X., Zi, S., Wu, L., 2021b, Gut microbiota protects honey bees (Apis melllifera L.) against polystyrene microplastic exposure risks: Journal of Hazardous Materials, v. 402, article 123828, accessed March 22, 2024, at https://doi.org/10.1016/j.jhazmat.2020.123828.
Wang, W., and Wang, J., 2018, Investigation of microplastics in aquatic environments—An overview of the methods used, from field sampling to laboratory analysis: Trends in Analytical Chemistry, v. 108, p. 195–202, accessed March 22, 2024, at https://doi.org/10.1016/j.trac.2018.08.026.
Wang, Y., Yang, Y., Liu, X., Zhao, J., Liu, R., and Xing, B., 2021c, Interaction of microplastics with antibiotics in aquatic environment—Distribution, adsorption, and toxicity: Environmental Science & Technology, v. 55, no. 23, p. 15579–15595. [Also available at https://doi.org/10.1021/acs.est.1c04509.]
Wang, Z.-M., Wagner, J., Ghosal, S., Bedi, G., and Wall, S., 2017, SEM/EDS and optical microscopy analyses of microplastics in ocean trawl and fish guts: Science of the Total Environment, v. 603–604, p. 616–626., accessed March 22, 2024, at https://doi.org/10.1016/j.scitotenv.2017.06.047.
Wardrop, P., Shimeta, J., Nugegoda, D., Morrison, P.D., Miranda, A., Tang, M., and Clarke, B.O., 2016, Chemical pollutants sorbed to ingested microbeads from personal care products accumulate in fish: Environmental Science & Technology, v. 50, no. 7, p. 4037–4044. [Also available at https://doi.org/10.1021/acs.est.5b06280.]
Watkins, L., Sullivan, P.J., and Walter, M.T., 2021, What you net depends on if you grab—A meta-analysis of sampling method’s impact on measured aquatic microplastic concentration: Environmental Science & Technology, v. 55, no. 19, p. 12930–12942. [Also available at https://doi.org/10.1021/acs.est.1c03019.]
Watts, A.J.R., Lewis, C., Goodhead, R.M., Beckett, S.J., Moger, J., Tyler, C.R., and Galloway, T.S., 2014, Uptake and retention of microplastics by the shore crab Carcinus maenas: Environmental Science & Technology, v. 48, no. 15, p. 8823–8830. [Also available at https://doi.org/10.1021/es501090e.]
Web of Science, 2024, Search--Results for microplastics (all fields): Web of Science Core Collection website, accessed January 5, 2024, at https://www.webofscience.com/wos/woscc/basick-search.
Weis, J.S., 2020, Aquatic microplastic research—A critique and suggestions for the future: Water, v. 12, no. 5, article 1475. [Also available at https://doi.org/10.3390/w12051475.]
Weis, J.S., and Palmquist, K.H., 2021, Reality check—Experimental studies on microplastics lack realism: Applied Sciences, v. 11, no. 18, article 8529, 16 p. [Also available at https://doi.org/10.3390/app11188529.]
Werbowski, L.M., Gilbreath, A.N., Munno, K., Zhu, X., Grbic, J., Wu, T., Sutton, R., Sedlak, M.D., Deshpande, A.D., and Rochman, C.M., 2021, Urban stormwater runoff—A major pathway for anthropogenic particles, black rubbery fragments, and other types of microplastics to urban receiving waters: ACS ES&T Water, v. 1, no. 6, p. 1420–1428. [Also available at https://doi.org/10.1021/acsestwater.1c00017.]
Wetherbee, G., Baldwin, A., and Ranville, J., 2019, It is raining plastic: U.S. Geological Survey Open-File Report 2019–1048, 1 p., accessed March 22, 2024, at https://doi.org/10.3133/ofr20191048.
Whitmire, S.L., and Bloem, S.J. van, 2017, Quantification of microplastics on national park beaches, 06/01/2015–05/31/2017: National Oceanic and Atmospheric Administration, Marine Debris Program report, prepared by Baruch Institute of Coastal Ecology & Forest Science, Clemson University under contract no. GSI–CU–1505, 28 p. [Also available at https://marinedebris.noaa.gov/sites/default/files/publications-files/Quantification_of_Microplastics_on_National_Park_Beaches.pdf.]
Williams, A.J., Grulke, C.M., Edwards, J., McEachran, A.D., Mansouri, K., Baker, N.C., Patlewicz, G., Shah, I., Wambaugh, J.F., Judson, R.S., and Richard, A.M., 2017, The CompTox chemistry dashboard—A community data resource for environmental chemistry: Journal of Cheminformatics, v. 9, article 61. [Also available at https://doi.org/10.1186/s13321-017-0247-6.]
Woodall, L.C., Sanchez-Vidal, A., Canals, M., Paterson, G.L.J., Coppock, R., Sleight, V., Calafat, A., Rogers, A.D., Narayanaswamy, B.E., and Thompson, R.C., 2014, The deep sea is a major sink for microplastic debris: Royal Society Open Science, v. 1, no. 4, article 140317. [Also available at https://doi.org/10.1098/rsos.140317.]
Woods, M.N., Stack, M.E., Fields, D.M., Shaw, S.D., and Matrai, P.A., 2018, Microplastic fiber uptake, ingestion and egestion rates in the blue mussel (Mytilus edulis): Marine Pollution Bulletin, v. 137, p. 638–645, accessed March 22, 2024, at https://doi.org/10.1016/j.marpolbul.2018.10.061.
Wong, C.S., and Coffin, S., 2021a, Standard operating procedures for extraction and measurement by Raman spectroscopy of microplastic particles in drinking water: California Water Boards, State Water Resources Control Board, 32 p., accessed June 24, 2022, at https://www.waterboards.ca.gov/drinking_water/certlic/drinkingwater/documents/microplastics/mcrplstcs_raman.pdf.
Wong, C.S., and Coffin, S., 2021b, Standard operating procedures for extraction and measurement by infrared spectroscopy of microplastic particles in drinking water: California Water Boards, State Water Resources Control Board, 30 p., accessed June 24, 2022, at https://www.waterboards.ca.gov/drinking_water/certlic/drinkingwater/documents/microplastics/mcrplstcs_ir.pdf.
Wong, J.K.H., Lee, K.K., Tang, K.H.D., and Yap, P.-S., 2020, Microplastics in the freshwater and terrestrial environments—Prevalence, fates, impacts and sustainable solutions: Science of the Total Environment, v. 719, article 137512. [Also available at https://doi.org/10.1016/j.scitotenv.2020.137512.]
Wright, S.L., Thompson, R.C., and Galloway, T.S., 2013, The physical impacts of microplastics on marine organisms—A review: Environmental Pollution, v. 178, p. 483–492, accessed March 22, 2024, at https://doi.org/10.1016/j.envpol.2013.02.031.
Wu, Y., Guo, P., Zhang, X., Zhang, Y., Xie, S., and Deng, J., 2019, Effect of microplastics exposure on the photosynthesis system of freshwater algae: Journal of Hazardous Materials, v. 374, p. 219–227. [Also available at https://doi.org/10.1016/j.jhazmat.2019.04.039.]
Xiong, X., Tu, Y., Chen, X., Jiang, X., Shi, H., Wu, C., and Elser, J.J., 2019, Ingestion and egestion of polyethylene microplastics by goldfish (Carassius auratus)—Influence of color and morphological features: Heliyon, v. 5, no. 12, article e03063. [Also available at https://pubmed.ncbi.nlm.nih.gov/32083206/.]
Xu, L.L., and Karlsson, A., 2019, How can science help save our marine environment?: Elsevier, accessed May 22, 2019, at www.elsevier.com/connect/how-can-science-help-save-our-marine-environment.
Xu, S., Ma, J., Ji, R., Pan, K., and Miao, A.-J., 2020, Microplastics in aquatic environments—Occurrence, accumulation, and biological effects: Science of the Total Environment, v. 703, article 134699. [Also available at https://doi.org/10.1016/j.scitotenv.2019.134699.]
Xue, N., Fang, Q., Pan, X., and Zhang, D., 2021, Distinct fungal plastisphere across different river functional zones—A watershed scale study: Science of the Total Environment, v. 752, article 141879. [Also available at https://doi.org/10.1016/j.scitotenv.2020.141879.]
Zangmeister, C.D., Radney, J.G., Benkstein, K.D., and Kalanyan, B., 2022, Common single-use consumer plastic products release trillions of sub-100 nm nanoparticles per liter into water during normal use: Environmental Science & Technology, v. 56, no. 9, p. 5448–5455. [Also available at https://doi.org/10.1021/acs.est.1c06768.]
Zantis, L.J., Carroll, E.L., Nelms, S.E., and Bosker, T., 2021, Marine mammals and microplastics—A systematic review and call for standardization: Environmental Pollution, v. 269, article 116142, 12 p., accessed October 31, 2023, at https://www.sciencedirect.com/science/article/pii/S0269749120368317.
Zettler, E.R., Mincer, T.J., and Amaral-Zettler, L.A., 2013, Life in the “plastisphere”—Microbial communities on plastic marine debris: Environmental Science & Technology, v. 47, no. 13, p. 7137–7146. [Also available at https://doi.org/10.1021/es401288x.]
Zhang, F., Wang, X., Xu, J., Zhu, L., Peng, G., Xu, P., and Li, D., 2019, Food-web transfer of microplastics between wild caught fish and crustaceans in East China Sea: Marine Pollution Bulletin, v. 146, p. 173–182. [Also available at https://doi.org/10.1016/j.marpolbul.2019.05.061.]
Zhang, Q., Xu, E.G., Li, J., Chen, Q., Ma, L., Zeng, E.Y., and Shi, H., 2020, A review of microplastics in table salt, drinking water, and air—Direct human exposure: Environmental Science & Technology, v. 54, no. 7, p. 3740–3751. [Also available at https://doi.org/10.1021/acs.est.9b04535.]
Zhu, X., Munno, K., Grbic, J., Werbowski, L.M., Bikker, J., Ho, A., Guo, E., Sedlak, M., Sutton, R., Box, C., Lin, D., Gilbreath, A., Holleman, R.C., Fortin, M.-J., and Rochman, C., 2021, Holistic assessment of microplastics and other anthropogenic microdebris in an urban bay sheds light on their sources and fate: ACS ES&T Water, v. 1, no. 6, p. 1401–1410. [Also available at https://doi.org/10.1021/acsestwater.0c00292.]
Ziajahromi, S., and Leusch, F.D.L., 2022, Systematic assessment of data quality and quality assurance/quality control (QA/QC) of current research on microplastics in biosolids and agricultural soils: Environmental Pollution, v. 294, article 118629. [Also available at https://doi.org/10.1016/j.envpol.2021.118629.]
Ziajahromi, S., Neale, P.A., Silveira, I.T., Chua, A., and Leusch, F.D.L., 2021, An audit of microplastic abundance throughout three Australian wastewater treatment plants: Chemosphere, v. 263, article 128294. [Also available at https://doi.org/10.1016/j.chemosphere.2020.128294.]
Zimmermann, L., Göttlich, S., Oehlmann, J., Wagner, M., and Völker, C., 2020, What are the drivers of microplastic toxicity? Comparing the toxicity of plastic chemicals and particles to Daphnia magna: Environmental Pollution, v. 267, article 115392. [Also available at https://doi.org/10.1016/j.envpol.2020.115392.]
Appendix 1. Comparison of microplastic analytical methods
Table 1.1.
Summary table of microplastic analyses and their advantages and limitations.[Symbols: X, times; ≥, greater than or equal to; <, less than; Methods: GC, gas chromatography; GPC, high-temperature gel-permeation chromatography; IR, infrared spectrometry; LC/MS, liquid chromatography-mass spectrometry; MS, mass spectrometry; Pyr-GC/MS, pyrolysis-gas chromatography-mass spectrometry; TD-GC/MS, thermal desorption gas chromatography-mass spectrometry; Units: µg/mL, micrograms per milliliter; µm, micrometer; mm, millimeter; nm, nanometer; Other: USGS, U.S. Geological Survey]
Method name | Methodology | Advantages | Limitations | References |
---|---|---|---|---|
Light microscopy counting (stereomicroscope, compound microscope)1 | Samples are visually examined at magnifications as great as 1,000X to identify, count, and physically characterize microplastics (particle size ≥ 100 µm). | Nondestructive, simple procedure, low technical expertise, and low startup costs. | Results are potentially biased, chemical classification not possible, high lower size limit, time consuming, and labor intensive. | Abbasi and others, 2017; Elkhatib and Oyanedel-Craver, 2020300 |
Scanning electron microscopy (optional addition of energy dispersive X-ray spectrometry)2 | High-energy beam of electrons interacts with sample surface to produce visual images
at magnifications as great as 30,000X to identify, count, and physically characterize
(particle size ≥ 10 µm). |
Microplastics < 100 µm can be visualized with higher resolution and views of surface textures for more accurate identification and confirmation of plastics versus natural organic debris. | Expensive, long sample preparation and analysis times, only capable of visual counting and physical characterization, since few plastics have an identifying energy dispersive X-ray spectrometry peak. | Kalčíková and others, 2017; Shim and others, 2017; Wagner and others, 2017; Wang and others, 2017; Qiu and others, 2016 |
Fluorescence microscopy with Nile Red staining1 | Staining with lipophilic fluorescent Nile Red dye followed by visual imaging under fluorescence microscope to identify and count microplastics (particle size ≥ 50 µm). | Reproducible quantitation, high sample throughput, low cost, and automated particle counting. | Requires complete elimination of natural organic material from sample to avoid false positive; limited ability to identify polymer types, and background fluorescence subtraction is subjective. | Elkhatib and Oyanedel-Craver, 2020; Erni-Cassola and others, 2017; Maes and others, 2017; Shim and others, 2016; Hidalgo-Ruz and others, 2012 |
Microscopy coupled Fourier-transform infrared spectroscopy4 | Produces spectra in response to IR light to identify, count, and chemically characterize microplastics (particle size ≥ 20 µm). | Capable of chemical classification and quantitation of polymers, particle counting can be automated, and extensive spectral libraries area available for polymer identification. | More expensive than microscopy and slow sample analysis. | Besseling and others, 2015; Käppler and others, 2016; Song and others, 2015; Qiu and others, 2016; Ivleva and others, 2017; Shim and others, 2017; Wagner and others, 2017; Zhao and others, 2017; Araújo and others, 2018; Cabernard and others, 2018; Uddin and others, 2020 |
Microscopy coupled Raman spectroscopy1 | Produces spectra in response to low-wavelength laser to identify, count, and chemically characterize microplastics (particle size ≥ 1 µm). | Detection of very small microplastics, capable of chemical classification and quantitation of polymers, particle counting can be automated, and extensive spectral libraries available for polymer identification. | More expensive than microscopy, slow throughput, tendency for spectral distortion at very small sizes, and slow sample analysis (unless machine learning software used). | Besseling and others, 2015; Käppler and others, 2016; Song and others, 2015; Qiu and others, 2016; Ivleva and others, 2017; Shim and others, 2017; Wagner and others, 2017; Zhao and others, 2017; Araújo and others, 2018; Cabernard and others, 2018; Uddin and others, 2020 |
Laser direct infrared spectroscopy1 | Uses laser-based chemical imaging and infrared to identify, count, and chemically characterize microplastics (particle size ≥ 10 µm). | Provides spectra in seconds for each particle, provides data on the abundance, size distribution, and type of plastics in each sample. More sensitive than other methods (fewer missed particles). | Difficulty resolving mixed polymers, expensive startup cost, not yet developed into a commonly applied method for microplastics aqueous samples. | Scircle and others, 2020 |
Flow cytometry (with or without fluorescent dye markers)2 | Enables high-precision particle detection by light scattering and (or) fluorescence of polymer structure, concomitant pigments, or added fluorescent dye markers (particle size ≥ 0.1 µm). | Identification of very small microplastics (nanoplastics), with high throughput and automated particle counting of aqueous samples with limited preparation requirements, some chemical classification is possible. | Mostly lab tested at this point, not yet developed into a commonly applied method for microplastics aqueous samples, best with few defined size populations. | Sgier and others, 2016; Summers and others, 2018; Kaile and others, 2020; Primpke and others, 2020 |
Quantitative nuclear magnetic resonance spectroscopy3 | Polymers are dissolved into deuterated solvent and quantified with 1H–NMR. | Capable of polymer characterization and quantification with limits of detection in the range of 19–21 µg/mL. | Complex interpretation, destructive of samples, does not count particles or determine sizes or shapes. | Peez and others, 2019; Peez and Imhof, 2020 |
Pyrolysis-gas chromatography-mass spectrometry (Pyr-GC/MS)2 | Pyrolysis of sample, separation of polymer types and other chemicals by GC, followed by detection with MS. | Low background contamination, simultaneous quantification of polymer types and sorbed chemicals at low limits of detection (nanoplastics). | Complex interpretation, limited polymers in MS database, destructive of samples, does not count particles or determine sizes or shapes. | Duemichen and others, 2014; Fischer and Scholz-Böttcher, 2017; Fischer and Scholz-Böttcher, 2019; Shim and others, 2017; Hermabessiere and others, 2018; Li, and others, 2018 |
Thermal desorption gas chromatography-mass spectrometry (TD-GC/MS)4 | Thermal desorption of sample, separation of polymer types and other chemicals by GC, followed by detection with MS. | Low background contamination, simultaneous quantification of polymer types and sorbed chemicals at low limits of detection (nanoplastics). | Complex interpretation, limited polymers in MS database, destructive of samples, does not count particles or determine sizes or shapes. | Duemichen and others, 2014; Fischer and Scholz-Böttcher, 2017; 2019Shim and others, 2017; Hermabessiere and others, 2018; Li, and others, 2018 |
Liquid chromatography-mass spectrometry (LC/MS)3 | Samples dissolved in solvent and molar mass distribution is measured. | High recoveries of selected polymers, detection can occur at low limits of detection. | Complex interpretation, not all polymer types can be analyzed by LC/MS, does not count particles or determine sizes or shapes. | Elert and others, 2017 |
High-temperature gel-permeation chromatography3 | Twofold density separation, extraction, and subsequent quantitation of polymers (by weight). | Characterizes polymer molecular weight, type, and bulk sample weight. | Complex interpretation, few polymer types have been analyzed by HT-GPC, does not count particles or determine shapes. | Hintersteiner and others, 2015 |
Portable handheld optical sensor4 | Uses laser light source at 635 nm with photodiode detector and charged-coupled device to record specular reflection signal and transmitted interference patterns (particle size 1 µm–5 mm). | Screens the type, the size, and the nonplanarity of microplastics in water and sediment. | Lab tested only on two polymer types. Unclear as to utility in the field and (or) effect of natural particles in suspension. | Asamoah and others, 2019 |
References Cited in Appendix 1
Abbasi, S., Keshavarzi, B., Moore, F., Delshab, H., Soltani, N., and Sorooshian, A., 2017, Investigation of microrubbers, microplastics and heavy metals in street dust—A study in Bushehr city, Iran: Environmental Earth Sciences, v. 76, article 798. [Also available at https://doi.org/10.1007/s12665-017-7137-0.]
Araújo, C.F., Nolasco, M.M., Ribeiro, A.M.P., and Ribeiro-Claro, P.J.A., 2018, Identification of microplastics using Raman spectroscopy—Latest developments and future prospects: Water Research, v. 142, p. 426–440. [Also available at https://doi.org/10.1016/j.watres.2018.05.060.]
Asamoah, B.O., Kanyathare, B., Roussey, M., and Peiponen, K.E., 2019, A prototype of a portable optical sensor for the detection of transparent and translucent microplastics in freshwater: Chemosphere, v. 231, p. 161–167. [Also available at https://doi.org/10.1016/j.chemosphere.2019.05.114.]
Besseling, E., Foekema, E.M., Franeker, J.A. van, Leopold, M.F., Kühn, S., Bravo Rebolledo, E.B., Heße, E., Mielke, L., Ijzer, J., Kamminga, P., and Koelmans, A.A., 2015, Microplastic in a macro filter feeder—Humpback whale Megaptera novaeangliae: Marine Pollution Bulletin, v. 95, no. 1, p. 248–252. [Also available at https://doi.org/10.1016/j.marpolbul.2015.04.007.]
Cabernard, L., Roscher, L., Lorenz, C., Gerdts, G., and Primpke, S., 2018, Comparison of Raman and Fourier transform infrared spectroscopy for the quantification of microplastics in the aquatic environment: Environmental Science & Technology, v. 52, no. 22, p. 13279–13288. [Also available at https://doi.org/10.1021/acs.est.8b03438.]
Duemichen, E., Braun, U., Senz, R., Fabian, G., and Sturm, H., 2014, Assessment of a new method for the analysis of decomposition gases of polymers by a combining thermogravimetric solid-phase extraction and thermal desorption gas chromatography mass spectrometry: Journal of Chromatography A, v. 1354, p. 117–128. [Also available at https://doi.org/10.1016/j.chroma.2014.05.057.]
Elert, A.M., Becker, R., Duemichen, E., Eisentraut, P., Falkenhagen, J., Sturm, H., and Braun, U., 2017, Comparison of different methods for MP detection—What can we learn from them, and why asking the right question before measurements matters?: Environmental Pollution, v. 231, p. 1256–1264. [Also available at https://doi.org/10.1016/j.envpol.2017.08.074.]
Elkhatib, D., and Oyanedel-Craver, V., 2020, A critical review of extraction and identification methods of microplastics in wastewater and drinking water: Environmental Science & Technology, v. 54, no. 12, p. 7037–7049. [Also available at https://doi.org/10.1021/acs.est.9b06672.]
Erni-Cassola, G., Gibson, M.I., Thompson, R.C., and Christie-Oleza, J.A., 2017, Lost, but found with Nile red—A novel method for detecting and quantifying small microplastics (1 mm to 20 μm) in environmental samples: Environmental Science & Technology, v. 51, no. 23, p. 13641–13648. [Also available at https://doi.org/10.1021/acs.est.7b04512.]
Fischer, M., and Scholz-Böttcher, B.M., 2017, Simultaneous trace identification and quantification of common types of microplastics in environmental samples by pyrolysis-gas chromatography-mass spectrometry: Environmental Science & Technology, v. 51, no. 9, p. 5052–5060. [Also available at https://doi.org/10.1021/acs.est.6b06362.]
Fischer, M., and Scholz-Böttcher, B.M., 2019, Microplastics analysis in environmental samples—Recent pyrolysis-gas chromatography-mass spectrometry method improvements to increase the reliability of mass-related data: Analytical Methods, v. 11, no. 18, p. 2489–2497. [Also available at https://doi.org/10.1039/C9AY00600A.]
Hermabessiere, L., Himber, C., Boricaud, B., Kazour, M., Amara, R., Cassone, A.-L., Laurentie, M., Paul-Pont, I., Soudant, P., Dehaut, A., and Duflos, G., 2018, Optimization, performance, and application of a pyrolysis-GC/MS method for the identification of microplastics: Analytical and Bioanalytical Chemistry, v. 410, no. 25, p. 6663–6676. [Also available at https://doi.org/10.1007/s00216-018-1279-0.]
Hidalgo-Ruz, V., Gutow, L., Thompson, R.C., and Thiel, M., 2012, Microplastics in the marine environment—A review of the methods used for identification and quantification: Environmental Science & Technology, v. 46, no. 6, p. 3060–3075. [Also available at https://doi.org/10.1021/es2031505.]
Hintersteiner, I., Himmelsbach, M., and Buchberger, W.W., 2015, Characterization and quantitation of polyolefin microplastics in personal-care products using high-temperature gel-permeation chromatography: Analytical and Bioanalytical Chemistry, v. 407, no. 4, p. 1253–1259. [Also available at https://doi.org/10.1007/s00216-014-8318-2.]
Ivleva, N.P., Wiesheu, A.C., and Niessner, R., 2017, Microplastic in aquatic ecosystems: Angewandte Chemie, v. 56, no. 7, p. 1720–1739. [Also available at https://doi.org/10.1002/anie.201606957.]
Kaile, N., Lindivat, M., Elio, J., Thuestad, G., Crowley, Q.G., and Hoell, I.A., 2020, Preliminary results from detection of microplastics in liquid samples using flow cytometry: Frontiers in Marine Science, v. 7, [Also available at https://doi.org/10.3389/fmars.2020.552688.]
Kalčíková, G., Alič, B., Skalar, T., Bundschuh, M., and Gotvajn, A.Ž., 2017, Wastewater treatment plant effluents as source of cosmetic polyethylene microbeads to freshwater: Chemosphere, v. 188, p. 25–31. [Also available at https://doi.org/10.1016/j.chemosphere.2017.08.131.]
Käppler, A., Fischer, D., Oberbeckmann, S., Schernewski, G., Labrenz, M., Eichhorn, K.J., and Voit, B., 2016, Analysis of environmental microplastics by vibrational microspectroscopy—FTIR, Raman or both?: Analytical and Bioanalytical Chemistry, v. 408, no. 29, p. 8377–8391. [Also available at https://doi.org/10.1007/s00216-016-9956-3.]
Li, J., Green, C., Reynolds, A., Shi, H., and Rotchell, J.M., 2018, Microplastics in mussels sampled from coastal waters and supermarkets in the United Kingdom: Environmental Pollution, v. 241, p. 35–44. [Also available at https://doi.org/10.1016/j.envpol.2018.05.038.]
Maes, T., Jessop, R., Wellner, N., Haupt, K., and Mayes, A.G., 2017, A rapid-screening approach to detect and quantify microplastics based on fluorescent tagging with Nile Red: Nature, v. 7, no. 1, article 44501. [Also available at https://doi.org/10.1038/srep44501.]
Peez, N., and Imhof, W., 2020, Quantitative 1H-NMR spectroscopy as an efficient method for identification and quantification of PVC, ABS and PA microparticles: Analyst, v. 145, no. 15, p. 5363–5371. [Also available at https://doi.org/10.1039/D0AN00879F.]
Peez, N., Janiska, M.-C., and Imhof, W., 2019, The first application of quantitative 1H NMR spectroscopy as a simple and fast method of identification and quantification of microplastic particles (PE, PET, and PS): Analytical and Bioanalytical Chemistry, v. 411, no. 4, p. 823–833. [Also available at https://doi.org/10.1007/s00216-018-1510-z.]
Primpke, S., Christiansen, S.H., Cowger, W., Frond, H. de, Deshpande, A., Fischer, M., Holland, E.B., Meyns, M., O’Donnell, B.A., Ossmann, B.E., Pttroff, M., Sarau, G., Scholz-Böttcher, B.M., and Wiggin, K.J., 2020, Critical assessment of analytical methods for the harmonized and cost-efficient analysis of microplastics: Applied Spectroscopy, v. 74, no. 9, p. 1012–1047. [Also available at https://doi.org/10.1177/0003702820921465.]
Qiu, Q., Tan, Z., Wang, J., Peng, J., Li, M., and Zhan, Z., 2016, Extraction, enumeration and identification methods for monitoring microplastics in the environment: Estuarine, Coastal and Shelf Science, v. 176, p. 102–109. [Also available at https://doi.org/10.1016/j.ecss.2016.04.012.]
Scircle, A., Cizdziel, J.V., Tisinger, L., Anumol, T., and Robey, D., 2020, Occurrence of microplastic pollution at oyster reefs and other coastal sites in the Mississippi Sound, USA—Impacts of freshwater inflows from flooding: Toxics, v. 8, no. 2, article 35. [Also available at https://doi.org/10.3390/toxics8020035.]
Sgier, L., Freimann, R., Zupanic, A., and Kroll, A., 2016, Flow cytometry combined with viSNE for the analysis of microbial biofilms and detection of microplastics: Nature Communications, v. 7, no. 1, article 11587. [Also available at https://doi.org/10.1038/ncomms11587.]
Shim, W.J., Hong, S.H., and Eo, S.E., 2017, Identification methods in microplastic analysis—A review: Analytical Methods, v. 9, no. 9, p. 1384–1391. [Also available at https://doi.org/10.1039/C6AY02558G.]
Shim, W.J., Song, Y.K., Hong, S.H., and Jang, M., 2016, Identification and quantification of microplastics using Nile Red staining: Marine Pollution Bulletin, v. 113, no. 1–2, p. 469–476. [Also available at https://doi.org/10.1016/j.marpolbul.2016.10.049.]
Song, Y.K., Hong, S.H., Jang, M., Han, G.M., Rani, M., Lee, J., and Shim, W.J., 2015, A comparison of microscopic and spectroscopic identification methods for analysis of microplastics in environmental samples: Marine Pollution Bulletin, v. 93, no. 1–2, p. 202–209. [Also available at https://doi.org/10.1016/j.marpolbul.2015.01.015.]
Summers, S., Henry, T., and Gutierrez, T., 2018, Agglomeration of nano-and microplastic particles in seawater by autochthonous and de novo-produced sources of exopolymeric substances: Marine Pollution Bulletin, v. 130, p. 258–267. [Also available at https://doi.org/10.1016/j.marpolbul.2018.03.039.]
Uddin, S., Fowler, S.W., and Saeed, T., 2020, Microplastic particles in the Persian/Arabian Gulf—A review on sampling and identification: Marine Pollution Bulletin, v. 154, article 111100. [Also available at https://doi.org/10.1016/j.marpolbul.2020.111100.]
Wagner, J., Wang, Z.-M., Ghosal, S., Rochman, C., Gassel, M., and Wall, S., 2017, Novel method for the extraction and identification of microplastics in ocean trawl and fish gut matrices: Analytical Methods, v. 9, no. 9, p. 1479–1490. [Also available at https://doi.org/10.1039/C6AY02396G.]
Wang, Z.-M., Wagner, J., Ghosal, S., Bedi, G., and Wall, S., 2017, SEM/EDS and optical microscopy analyses of microplastics in ocean trawl and fish guts: Science of the Total Environment, v. 603–604, p. 616–626. [Also available at https://doi.org/10.1016/j.scitotenv.2017.06.047.]
Zhao, S., Danley, M., Ward, J.E., Li, D., and Mincer, T.J., 2017, An approach for extraction, characterization and quantitation of microplastic in natural marine snow using Raman microscopy: Analytical Methods, v. 9, no. 9, p. 1470–1478. [Also available at https://doi.org/10.1039/C6AY02302A.]
Conversion Factors
International System of Units to U.S. customary units
Abbreviations
DDT
dichloro-diphenyl-trichloroethane
EPA
U.S. Environmental Protection Agency
FTIR
Fourier-transform infrared spectroscopy
LC/MS
liquid chromatography-mass spectrometry
micro-FTIR
Fourier-transform infrared spectroscopy coupled with microscopy
micro-Raman
Raman microscopy
NIST
National Institute of Standards and Technology
NOAA
National Oceanic and Atmospheric Administration
PTFE
polytetrafluoroethylene
PVC
polyvinyl chloride
Pyr-GC/MS
pyrolysis gas chromatography-mass spectrometry
QA/QC
quality assurance and quality control
SRM(s)
standard reference material(s)
TD-GC/MS
thermal desorption gas chromatography-mass spectrometry
USGS
U.S. Geological Survey
>
greater than
<
less than
+
plus
For additional information, contact:
Program Coordinator, Environmental Health Program
U.S. Geological Survey
12201 Sunrise Valley Drive
Reston, VA 20192
Publishing support provided by U.S. Geological Survey Science Publishing Network, Reston and Baltimore Publishing Service Centers
Disclaimers
Any use of trade, firm, or product names is for descriptive purposes only and does not imply endorsement by the U.S. Government.
Although this information product, for the most part, is in the public domain, it also may contain copyrighted materials as noted in the text. Permission to reproduce copyrighted items must be secured from the copyright owner.
Suggested Citation
Iwanowicz, D.D., Baldwin, A.K., Barber, L.B., Blazer, V.S., Corsi, S.R., Duris, J.W., Fisher, S.C., Focazio, M., Janssen, S.E., Jasmann, J.R., Kolpin, D.W., Kraus, J.M., Lane, R.F., Lee, M.E., McSwain, K.B., Oden, T.D., Reilly, T.J., and Spanjer, A.R., 2024, Integrated science for the study of microplastics in the environment—A strategic science vision for the U.S. Geological Survey: U.S. Geological Survey Circular 1521, 54 p., https://doi.org/10.3133/cir1521.
ISSN: 2330-5703 (online)
ISSN: 1067-084X (print)
Publication type | Report |
---|---|
Publication Subtype | USGS Numbered Series |
Title | Integrated science for the study of microplastics in the environment—A strategic science vision for the U.S. Geological Survey |
Series title | Circular |
Series number | 1521 |
ISBN | 978-1-4113-4554-6 |
DOI | 10.3133/cir1521 |
Year Published | 2024 |
Language | English |
Publisher | U.S. Geological Survey |
Publisher location | Reston, VA |
Contributing office(s) | Office of the AD Ecosystems |
Description | vi, 54 p. |
Online Only (Y/N) | N |
Additional Online Files (Y/N) | N |
Google Analytic Metrics | Metrics page |