Guide to Per- and Polyfluoroalkyl Substances (PFAS) Sampling within Natural Resource Damage Assessment and Restoration
Links
- Document: Report (2.5 MB pdf) , HTML , XML
- Download citation as: RIS | Dublin Core
Abstract
Per- and polyfluoroalkyl substances (PFAS) are synthetic chemicals with a nondegradable fluorinated carbon backbone that have been incorporated in countless industrial and commercial applications. Because PFAS are nondegradable, they have been detected in all environmental media, indicating extensive global contamination. The unique physiochemical properties of PFAS and their complex interactions with environmental matrices create a great challenge for researchers when selecting site-specific sample matrices, sampling logistics, various analytical methods, and data interpretation. The widespread contamination and the potential toxicity of PFAS to human and environmental health have resulted in the proposed designation of two commonly used PFAS as hazardous substances, which may prompt new requirements for reporting, regulatory action, and site cleanup. For researchers involved in natural resource damage assessment efforts, understanding the multifaceted dynamics of the environmental fate and transport of PFAS will be essential for appropriate sample collections, analyses, and data interpretation. This guide aims to provide fundamental concepts and considerations involved with environmental sampling for PFAS during site assessments.
1. Introduction
Per- and polyfluoroalkyl substances (PFAS) are a large, diverse group of synthetic chemicals that have received considerable public attention because of the growing concerns regarding their impact on human and environmental health. This has resulted in PFAS being a priority subject of State and Federal strategic plans, new rules, and regulatory action in the United States and globally. Under the Comprehensive Environmental Response, Compensation, and Liability Act (CERCLA) of 1980 the U.S. Environmental Protection Agency (EPA) is proposing to designate two commonly used PFAS, perfluorooctanesulfonic acid (PFOS) and perfluorooctanoic acid (PFOA), as hazardous substances. If these compounds are classified as hazardous substances, this may lead to spill reporting requirements, site cleanup, and remedial efforts (depending on site-specific conditions). Therefore, the purpose of this document is to introduce key PFAS concepts to personnel involved with Natural Resource Damage Assessment and Restoration (NRDAR) activities. This guide provides a brief overview of PFAS nomenclature and physiochemical properties (sec. 1), production, use, and regulatory status (sec. 1), environmental sources (sec. 1), environmental fate and transport (sec. 2), baseline considerations (sec. 3), response considerations (sec. 4), ephemeral data (sec. 5), restoration considerations (sec. 6), sampling techniques and collection preparation considerations (sec. 7), sampling design considerations (sec. 8), analytical methods (sec. 9), PFAS concentrations in environmental media (sec. 10), and a summary of key considerations (sec. 11). Table 1 provides the name and abbreviation for individual PFAS used throughout this document.
Nomenclature and Physicochemical Properties
PFAS are a complex class of structurally diverse synthetic compounds that consist of different physical and chemical properties (Buck and others, 2011; Cousins and others, 2020). There are at least nine definitions of PFAS provided by several organizations that differ from being very broad (carbon + fluorine atoms) to highly descriptive (branched; Hammel and others, 2022); however, PFAS generally contain a tail or a carbon chain (C) of varying length (C3–14) and fluorination attached to a head containing a charged functional group (for example, oxygen, hydrogen, nitrogen, carboxylates, sulfonates; fig. 1). PFAS with a fully fluorinated carbon chain (backbone; where the hydrogen atoms are entirely replaced by fluorine atoms) are known as perfluoroalkyl substances, whereas PFAS with carbon chains that are partially fluorinated are known as polyfluoroalkyl substances (fig. 1).
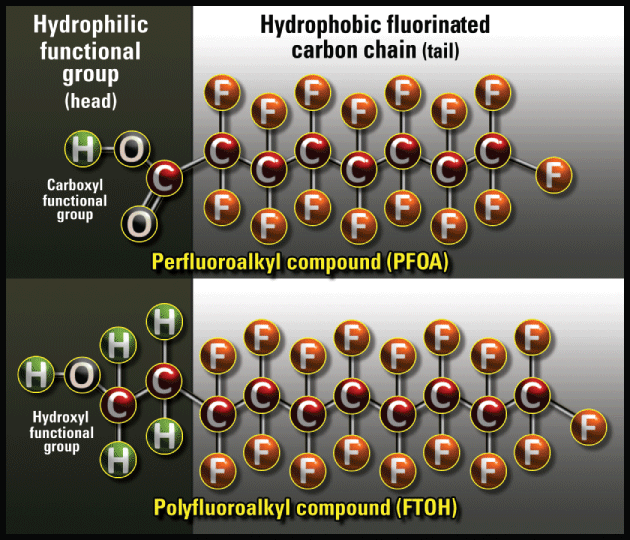
General molecular structure of a fully fluorinated perfluoroalkyl and a partially fluorinated polyfluoroalkyl substance (for example, a perfluoroalkyl compound and fluorotelomer alcohols, respectively). Figure modified from Espartero and others (2022).
The PFAS family has been estimated to include nearly 16,000 (with and without explicit chemical structures) chemical substances (EPA, 2023a). A few key publications discuss in depth the nomenclature and terminology of PFAS classifications (Buck and others, 2011; Cousins and others, 2020; Organisation for Economic Cooperation and Development, 2021; Wang and others, 2021); however, the Interstate Technology Regulatory Council (ITRC) has summarized the diverse variety of PFAS properties into a family tree consisting of two primary classes: polymers and nonpolymers (ITRC, 2021b). The nonpolymer class consists of two subclasses: the perfluoroalkyl and polyfluoroalkyl substances; furthermore, the group of perfluoroalkyl acids (PFAA)—such as perfluoroalkyl carboxylic acids (PFCA: such as PFOA) and perfluoroalkane sulfonic acids (PFSA; such as PFOS)—tend to be classified as either long-chain or short-chain compounds. The short-chain PFCA refers to those compounds with seven or fewer carbons of which six or less are perfluorinated, whereas their long-chain counterparts contain eight or more carbons of which seven or more are perfluorinated. Additionally, the short-chain PFSAs are those with five or fewer carbons that are perfluorinated, whereas the long-chain PFSAs are considered those with six or more carbons that are perfluorinated. Tables 1 and 2 summarize the nomenclature and physiochemical characteristics of the PFAS included in the widely accepted EPA and American Society for Testing and Materials (ASTM) methods further discussed in section 10.
Table 1.
List of names, abbreviations, class and group information for individual alkyl substances (PFAS) commonly included in monitoring efforts.[CASRN, Chemical Abstracts Service Registry Number]
Table 2.
Available partitioning coefficients for the individual per- and polyfluoroalkyl substance (PFAS) analytes included in commercially available laboratory methods.[CASRN, Chemical Abstracts Service Registry Number; g/mol, gram per mole; L/kg, liter per kilogram; --, no data; >, greater than; ±, plus or minus; <, less than]
Analyte abbreviation (table 1) | CASRN | Carbon chain length | Molecular weight (g/mol) | Acid dissociation (pKa) | Log octanol-air (log Koa) | Log octanol-water (log Kow) | Soil adsorption (sorption coefficient; (Kd or Koc) | Organic carbon (log Koc; L/kg) | Log albumin/water (log Kaw) |
---|---|---|---|---|---|---|---|---|---|
PFBA | 375-22-4 | 4 | 214.04 | 0.08a | 2.43b | 2.82c | 58.43b | 2.17a | 2.52d |
PFPeA | 2706-90-3 | 5 | 264.0459 | 0.569e | 4.99b | 3.43c | 269.9b | 2.43b | -- |
PFHxA | 307-24-4 | 6 | 314.054 | −0.16a | 5.24b | 4.06c | 1,247b | 2.06a | 3.43d |
PFHpA | 375-85-9 | 7 | 364.06 | −2.29f | 5.48b | 4.67c | 5,761b | 2.04a | 4.02d |
PFOA | 335-67-1 | 8 | 414.07 | −0.5a | 5.73b | 4.81f | 2.66´104 b | 2.06a | 4.20d |
PFNA | 375-95-1 | 9 | 464.08 | −0.21a | 5.98b | 5.92c | 1.23´105 b | 2.39a | 4.32d |
PFDA | 335-76-2 | 10 | 514.086 | −0.17a | -- | -- | -- | 2.79a | 4.73d |
PFUnDA | 2058-94-8 | 11 | 564.09 | −0.17a | -- | -- | -- | 3.30a | 4.60d |
PFDoDA | 307-55-1 | 12 | 614.1 | −0.17a | -- | -- | -- | 3.65–5.6g | -- |
PFTrDA | 726-94-8 | 13 | 664.11 | -- | -- | -- | -- | 3.71–5.2g | -- |
PFTeDA | 376-06-7 | 14 | 714.11 | −0.21g | -- | -- | -- | 3.68–5.2g | -- |
FBSA | 30334-69-1 | 4 | 299.111 | 5.98h | 4.73b | 3.72b | 2,789b | 3.45b | -- |
FhxSA | 41997-13-1 | 6 | 399.13 | -- | -- | -- | -- | -- | -- |
PFOSA | 754-91-6 | 8 | 499.14 | 6.24a | 5.70b | 7.58b | 1.27´106 b | 4.07–6.0g | -- |
HFPO-DA | 13252-13-6 | 6 | 330.053 | −0.77–2.8g | 5.74b | 3.6c | 118.8b | 2.08b | 3.06d |
N-MeFOSE | 24448-09-7 | 11 | 557.23 | -- | -- | -- | -- | -- | -- |
N-MeFOSA | 31506-32-8 | 9 | 513.169 | -- | -- | -- | -- | -- | -- |
N-MeFOSAA | 2355-31-9 | 11 | 571.21 | 3.92g | -- | -- | -- | 3.11–4.6g | -- |
N-EtFOSA | 4151-50-2 | 10 | 527.2 | 9.5h | -- | >6.8h | -- | 3.9 (±0.3)g | -- |
N-EtFOSAA | 2991-50-6 | 12 | 585.24 | 3.92g | -- | -- | -- | 3.23-4.8g | -- |
N-EtFOSE | 1691-99-2 | 12 | 571.25 | -- | -- | -- | -- | 3.95g | -- |
PFBS | 375-73-5 | 4 | 300.1 | 0.14a | 5.64b | 3.9c | 221.6b | 2.06a | 3.20d |
PFPeS | 2706-91-4 | 5 | 350.11 | -- | 5.89b | 3.38b | 1,024b | 3.01b | -- |
PFHxS | 355-46-4 | 6 | 400.12 | 0.14a | 6.13b | 5.17c | 4,726b | 2.28a | 4.81d |
PFHpS | 375-92-8 | 7 | 450.12 | -- | 6.38b | 5.31b | 2.19´104 b | 4.34b | -- |
PFOS | 1763-23-1 | 8 | 500.13 | 0.14a | -- | 6.3c | -- | 3.14a | 4.67d |
PFNS | 68259-12-1 | 9 | 550.14 | -- | -- | -- | -- | -- | -- |
PFDS | 335-77-3 | 10 | 600.15 | 0.14g | -- | -- | -- | 3.53–4.03g | -- |
4:2 FTS | 757124-72-4 | 6 | 328.15 | -- | -- | -- | -- | -- | -- |
6:2 FTS | 27619-97-2 | 8 | 428.17 | 1.31i | 5.39b | 3.85b | 1.53´104 b | 2.2–4.9g | -- |
8:2 FTS | 39108-34-4 | 10 | 529.18 | 1.32i | -- | -- | -- | 3.77g | -- |
NaDONA | 919005-14-4 | 7 | 378.07 | -- | -- | -- | -- | 1.98g | -- |
11Cl-PF3OudS | 763051-92-9 | 10 | 632.6 | -- | -- | -- | -- | 4.47g | -- |
9Cl-PF3ONS | 756426-58-1 | 8 | 532.58 | <1.0g | -- | -- | -- | 3.73g | -- |
3:3 FTCA | 356-02-5 | 6 | 242.09 | 4.18j | -- | -- | -- | -- | -- |
5:3 FTCA | 914637-49-3 | 8 | 342.11 | -- | -- | -- | -- | -- | -- |
7:3 FTCA | 812-70-4 | 10 | 442.12 | 4.22j | -- | -- | -- | -- | -- |
6:2 FTCA | 120885-29-2 | 7 | 363.05 | 2.82g | -- | -- | -- | 0.75g | -- |
8:2 FTCA | 27854-31-5 | 10 | 478.1 | -- | -- | -- | -- | -- | -- |
10:2 FTCA | 53826-13-4 | 12 | 578.117 | -- | -- | -- | -- | -- | -- |
6:2 FtUCA | 70887-88-6 | 8 | 358.081 | -- | -- | -- | -- | -- | -- |
FOUEA | 70887-84-2 | 10 | 458.1 | -- | -- | -- | -- | -- | -- |
PFDoS | 79780-39-5 | 12 | 700.16 | -- | -- | -- | -- | -- | -- |
NFDHA | 151772-58-6 | 5 | 296.04 | -- | -- | -- | -- | -- | -- |
PFEESA | 113507-82-7 | 4 | 316.1 | -- | -- | -- | -- | -- | -- |
PFMPA | 377-73-1 | 4 | 230.04 | -- | -- | -- | -- | -- | -- |
PFMBA | 863090-89-5 | 5 | 280.045 | -- | -- | -- | -- | -- | -- |
PFTA | 376-06-7 | 14 | 714.11 | -- | -- | -- | -- | -- | -- |
PfecHS | 646-83-3 | 8 | 462.13 | -- | -- | -- | -- | -- | 4.55d |
PFAS are considered amphipathic since they contain both hydrophilic and hydrophic properties. Many PFAS have a functional group, referred to as the head, attached to the fluorinated carbon chain, referred to as the tail (fig. 1). The head exhibits hydrophilic (water-loving) characteristics whereas the tail exhibits both hydrophobic (water repelling) and oleophobic/lipophilic (oil/fat-repelling) properties. The carbon-fluorine bond is considered one of the strongest in nature. This bond makes PFAS more chemically and thermally stable, allowing some PFAS to be highly resistant to environmental transformation including biodegradation, photooxidation, photolysis, and hydrolysis (Buck and others, 2011). These durable chemicals contain properties that include oil, water, temperature, chemical, fire resistance, and electrical insulating properties. It is these properties that make PFAS appealing for an array of industrial and consumer applications including textiles, leather, household goods, medical devices, inks, paper and food packaging, cosmetics, electronics, oil production, mining, pesticide formulations, outdoor gear, and apparel, and firefighting foams. The chemical durability and stability of PFAS renders them metabolically inert, low degradability, environmentally persistent and easily absorbed by organisms (Lau and others, 2007; Wang and others, 2017). It is these physicochemical characteristics that renders PFAS as effective materials in industrial and commercial applications but also renders them environmentally persistent, bioaccumulative, and toxic. Their chemical stability and extensive usage have resulted in the detection of these compounds in the environment, wildlife, humans, and food items (ATSDR, 2021; Fiedler and others, 2022; Sims and others, 2022).
Per- and Polyfluoroalkyl Substances (PFAS) Production, Historical Use, Current Use, and Regulatory Status
Development and synthesis of per- and polyfluoroalkyl substances (PFAS) began in 1938 with the discovery of polytetrafluoroethylene (PTFE). Commercial sales followed with the introduction of the Teflon (1949) and Scotchgard (1956) by DuPont and 3M, respectively. In the 1960s, aqueous film-forming foam (AFFF) fire suppressants were developed, and cookware and food packaging containing PFAS were approved for use (3M, 2021; Agency for Toxic Substances and Disease Registry [ATSDR], 2021). Total global emissions of perfluoroalkyl carboxylates (PFCAs; more information is in the section “Nomenclature and Physicochemical Properties”) between 1951 and 2015 are estimated to be between 2,610 and 21,400 tonnes with an additional 20–6,420 tonnes projected to be emitted from 2016 to 2030 (Wang and others, 2014). Although usage and production of two long-chain PFAS (PFOS and PFOA) have decreased in the United States and other countries, usage and production has increased in China, which continues to be a major producer, exporter, and user of these compounds (Li and others, 2015; ATSDR, 2021). Currently, the global manufacturing of PFAS consists of about (~) 250 facilities worldwide (Koll, 2022).
In early 2000, the EPA invited eight leading companies in the fluorochemical manufacturing industry to participate in the voluntary PFOA (and long-chain PFAS) Stewardship Program to reduce and eventually eliminate facility emissions and the use of related chemicals in product contents. Most of the companies involved stopped manufacturing and importing long-chain PFAS and transitioned to alternative products. As a result, industrial releases of the long-chain compounds in the United States have declined or have been eliminated, with the last report of PFOS being manufactured in 2002 (EPA, 2023b). It is important to note that PFOA and PFOS may still be produced domestically, imported, or used by those companies that are not participating in the voluntary program. Declines in emissions have also been reported in most industrialized nations that have eliminated production of PFOA and PFOS (Li and others, 2015; ATSDR, 2021). Additional efforts to phase out the domestic use and production of PFAS include the voluntarily phase out of certain long-chain, for example PFOS and PFOA, and short-chain, such as 6:2 fluorotelomer alcohols [FTOH], PFAS in food contact applications by the U.S. Food and Drug Administration (FDA, 2016, 2020). The EPA’s regulatory approach for short-chain length telomers and alternatives requires testing under the Toxic Substances Control Act (15 U.S.C. 2601–2692) to provide an adequate understanding of their fate and effects before their use. Since 2006, 191 new PFAS chemicals have been regulated under the Toxic Substances Control Act through the significant new use rules.
A proposal to designate PFOS and PFOA (including their salts and structural isomers) as hazardous substances under the CERCLA (42 U.S.C. §9601 et seq.) was published in the Federal Register in September of 2022. If officially listed as CERCLA hazardous substances, PFOS and PFOA could be included in Natural Resource Damage Assessments lead by Natural Resource Trustees. In May 2022, the EPA added five PFAS (HFPO-DA, PFOS, PFOA, PFNA, PFHxS) to a list of human-health risk-based values for site cleanups. These Regional Screening Levels are used by the EPA to determine if response or remediation activities are needed at contaminated sites. In January 2023, the EPA proposed significant new use rules to prevent resuming the manufacturing, importing, or use of an estimated 300 inactive PFAS that have not been used in the United States since 2006 without EPA review and risk determination (EPA, 2023c). In February 2023, the EPA announced the availability of $2 billion in Bipartisan Infrastructure Law (Public Law–58) funding for PFAS and emerging contaminants in drinking water. These funds will be available to small, rural, or disadvantaged communities to promote access to safe, clean drinking water. In March 2023, the EPA published the proposed National Primary Drinking Water Regulation in the Federal Register. The proposed new regulation will establish legally enforceable maximum contaminant levels (MCLs) for six PFAS (PFOA, PFOS, PFNA, PFHxS, PFBS, HFPO-DA) in drinking water. The proposed enforceable MCLs for PFOA and PFOS are 4.0 parts per trillion (pptr) for each. The enforceable limit for the combination of PFNA, PFHxS, PFBS, and HFPO-DA uses the hazard index approach to determine if the combined concentrations of these four PFAS pose a potential risk (EPA, 2023d). Health-based, nonenforceable maximum contaminant level goals (MCLGs) have also been proposed for these six PFAS. The MCLG for PFOA and PFOS is zero, whereas the hazard index approach is used for PFNA, PFHxS, PFBS, and HFPO-DA.
This guide is not meant to be a comprehensive literature review but instead highlights important properties and characteristics for PFAS and environmental media that may dictate the environmental fate, transport, and effects of PFAS. This guide is meant to give a broad overview on key concepts, nomenclature, and considerations for determining which environmental media to collect, sampling techniques, analytical methodology, PFAS concentrations in environmental media, and available guidance values and screening levels. For more in-depth detail on each of the topics covered in this document, the reader is highly encouraged to review the reference list, current literature, and links to resources provided.
Environmental Sources
There are countless applications and products containing PFAS that can contribute to environmental sources. Yet, there are still tremendous data gaps related to the scale, scope, and severity of direct and indirect releases of PFAS leading to environmental contamination (Salvatore and others, 2022). In the absence of these data, a presumptive approach suggests PFAS contamination is primarily from (1) industrial facilities that produce or use PFAS, for example metal platers using PFAS mist suppressants [such as PFOS, F-53B], automotive/metal stamping, and unspecified manufacturing); (2) fluorinated AFFF discharge sites (for example airports, military installations, and firefighting training areas); and (3) sites containing PFAS waste materials (Salvatore and others, 2022; fig. 2). Additional sources include landfills, wastewater treatment plants (WWTPs), biosolids, oil refineries, paper industries using PFAS treated paper for stain and oil proofing, paint manufacturing, tanneries using PFAS for waterproofing, and dry cleaners and laundromats (Liu and others, 2019; Helmer and others, 2022).
As of 2023, industries are now required to report the release of about 189 PFAS to the Toxics Release Inventory. Because of the reporting threshold (100 pounds per compound), it is likely that releases may be underreported. The 2021 Toxics Release Inventory reporting indicated the quantity of PFAS managed as waste increased by 59 percent (483,000 pounds) since 2020 (EPA, 2023e). This increase was largely driven by one facility in the chemical manufacturing sector that reported recycling of perfluoroocyl iodide which was not reportable in 2020. Most PFAS waste is managed by the hazardous waste management, chemical manufacturing, and plastics and rubber sectors the (EPA, 2023e). Similarly, releases of PFAS increased approximately five-fold from 2020 to 2021. This increase was mainly driven by the 2021 release of 68,500 pounds of PFAS to a landfill by the hazardous waste management sector (EPA, 2023e). The hazardous waste management sector reported 82 percent of all PFAS releases for 2021. In 2022, 93 percent of the total releases of PFAS were emitted to the land (EPA, 2023f). Major chemical manufacturing and industrial sources include those that produce textiles, leather, apparel, paper and packaging, photolithography, mining, metal plating, medical and household products, electronics, energy and oil drilling, food packaging and processing, personal-care products, aviation, construction materials, and biocides. The waste management sector includes WWTPs and landfills where diffuse releases of PFAS occur during disposal.

Environmental fate and transport of per- and polyfluoroalkyl substances (PFAS). PFAS can be from direct and indirect releases or emissions of PFAS from producers or industrial facilities manufacturing PFAS (outlined in red). Primary sources (outlined in orange) include consumer goods, PFAS-containing firefighting foams, landfills, and wastewater treatment plants. Secondary sources of PFAS (outlined in yellow) exposures include surface water, atmospheric deposition, agricultural crops, and foods (livestock, poultry). Receptors of PFAS contamination include wildlife, fish, birds, and humans. Illustration by Mike Hamblin, Hamblin Art.
2. Environmental Fate and Transport
PFAS have been coined the “forever chemicals” because of their resistance to biodegradation, photoxidation, direct photolysis, and hydrolysis. These chemicals are environmentally persistent, are easily transported through water and long-distance atmospheric transport and deposition, can contaminate groundwater and accumulate in biota, especially top predators in the food chain via bioaccumulation and biomagnification. The environmental fate and transport potential of PFAS is strongly affected by the complex interaction between the physicochemical properties of PFAS with the unique and variable properties of the natural environment. In multiple environmental compartments and systems (air, water, sediment), PFAS surfactants readily partition to various interfaces (for example surface-water interface, air-water interface [AWI]), thus playing an important role in the adsorption and transport of PFAS (Guo and others, 2023). Understanding the unique physical and chemical characteristics, fate, and transport of PFAS may be useful for determining the source, pathway, and subsequent exposure of natural resources and for developing assessment studies to determine injury to natural resources and the services they provide. This section briefly describes some of the primary physiochemical properties of PFAS and the media that influence fate and transport. Table 2 provides available partitioning coefficients.
Atmospheric Transport and Deposition
PFAS and their precursors are introduced into the atmosphere from many sources and physical mechanisms, including direct and fugitive emissions from industrial and commercial plants, AFFF releases, and the volatilization from landfills and WWTPs (Ahrens and others, 2011a; Roth and others, 2020; D’Ambro and others, 2021; Pfotenhauer and others, 2022). Studies have indicated PFAS-treated household and personal-care products (particularly the FTOHs) can contribute substantially to indoor ambient air and may represent a dominant direct route for human exposure (Morales-McDevitt and others, 2021); moreover, inhalation of gaseous PFAS may be an important source of firefighter exposure during the handling of AFFF, for example during firefighting, routine training or tank maintenance (Laitinen and others, 2014; Roth and others, 2020).
The atmosphere is not necessarily considered a reservoir for the long-term accumulation of PFAS but rather provides a medium for the short- and long-range transport of the gas and particulate phases of PFAS (Thackray and others, 2020). Atmospheric transport and wet and dry deposition are considered major contributors to the environmental transport and contamination of PFAS in aquatic and terrestrial environments, particularly remote and rural areas with fewer anthropogenic sources (Giesy and others, 2010). In particular, the volatile fluorotelomer compounds are readily transported through the atmosphere, and once oxidized, they serve as a secondary source of PFAS deposition (Faust, 2023). Models revealed dry deposition accounts for ~35 percent of global deposition, whereas wet deposition (precipitation) is considered the primary means for removal of highly water-soluble short-chain PFCAs in the atmosphere, although the deposition rates may be variable depending on location and precipitation rates. About 25 percent of the global releases of fluorotelomers are deposited as PFCAs with PFOA accounting for 4 percent annually (Thackray and others, 2020). Farther from direct emission sources, fluorotelomer degradation becomes the most important source of PFCAs. In the midlatitudes of the Northern Hemisphere, PFOA is deposited more than any other PFCA (Thackray and others, 2020). Thackray and others (2020) noted a continuous supply of PFCAs to the ocean even if direct sources of emissions cease because of the degradation of precursors over temperate oceans. PFAS contributions and reservoirs are largely uncertain, but they can remain in the atmosphere for decades; for example, production of PFOS in the United States was phased out in 2002, yet it is still observed as the dominant compound in the atmosphere in some areas of the United States and globally (Zhou and others, 2021; Saini and others, 2023). Some areas of the globe demonstrated an increasing trend of ambient PFOS, indicating a constant input from direct and indirect sources.
Soil and Sediment
Releases of PFAS from contaminated runoff, spills, or atmospheric deposition can infiltrate the ground surface and migrate through the vadose zone and into groundwater (Brusseau and others, 2020). As such, sediments and soils tend to be sinks for many organic compounds including PFAS and long-term sources of groundwater contamination. Environmental degradation in soil and sediment is considered negligible based on the chemical stability and biodegradation resistance of PFAS. Fate and transport of PFAS in subsurface environments are largely controlled by several processes, including adsorption to the solid-water interface, adsorption to the AWI, and the partitioning or adsorption to nonaqueous phase liquid-water interface (NWI; Higgins and Luthy, 2006; Guelfo and Higgins, 2013; Lyu and others, 2022). Prediction of PFAS in the solid-water interface is often modeled using the solid-water partition coefficient (Kd) and the organic carbon normalized distribution coefficient (Koc). PFAS concentration in soil porewater is relatively low because of the hydrophobic and oleophobic properties of PFAS resulting in the higher accumulation potential at the AWI in the vadose zone. Adsorption of PFAS at the AWI can contribute ~50 percent of the total mass retained in unsaturated porous media (Lyu and others, 2018; Lyu and others, 2022). To understand the transport and adsorption at the AWI, the AWI partition coefficient (Kai) can be used. At the NWI, sorption of PFAS to solids depends on the chain length and their concentrations. Experimental studies determined the PFAS adsorption at the NWI may contribute as much as 77 percent of PFOS and PFOA retention (Van Glubt and Brusseau, 2021) and can be predicted using the NWI adsorption coefficient (Ki); however, for substantial effects on nonaqueous phase liquid solubilization and mobilization, PFAS generally are only at high concentrations (100s of milligrams per liter [mg/L]) at contaminated sites such as those observed during AFFF release scenarios.
These processes are primarily influenced by the soil characteristics (for example organic carbon content, dry density) and the physiochemical properties (for example functional groups, chain length, molecular weight, structure, hydrophobicity) of PFAS (Higgins and Luthy, 2006; Ahrens and others, 2011b; Brusseau and others, 2020; Lyu and others, 2022; Radi and others, 2022). Adsorption and desorption in soil is dictated by the interaction between the soil organic carbon and the hydrophobic fluorinated carbon tail of PFAS (Mahinroosta and Senevirathna, 2020; Radi and others, 2022); for instance, significant correlations have been found between the organic carbon content and dry density of sediment for all PFAS investigated (Ahrens and others, 2011b). Although partitioning may be different for all compounds and sediments suggesting other mechanisms and factors (for example electrostatic interactions, salinity, temperature, pH) may influence sorption and transport (Higgins and Luthy, 2006; Ahrens and others, 2011b). Therefore, multiple soil properties should be measured to help understand and predict the adsorption and transport mechanisms of PFAS within different soil types. The interaction between geosorbents (such as clay particles) in the soil with the polar functional group of the PFAS head can also considerably influence sorption mechanisms. There is evidence that PFAS with PFCAs have a strong adsorption tendency compared to the sulfonic acids functional group (Radi and others, 2022), although other evidence suggests the larger molecular size and more hydrophobic sulfonate moiety (PFSAs) have a stronger sorption affinity to soil/sediments than PFCAs (Higgins and Luthy, 2006; Du and others, 2014; Li and others, 2019). Sediments are considered important sinks for PFAS, especially the long-chain PFAS (greater than or equal to [≥] C10) that tend to bind more readily to suspended particles, whereas the shorter-chain (less than or equal to [≤] C7) were more likely to be dissolved in the aqueous phase (Prevedouros and others, 2006; Ahrens and others, 2011b; Chen and others, 2019).
The dominant structural feature of PFAS that influences sorption potential is the perfluorocarbon chain length (Higgins and Luthy, 2006). Shorter-chain PFAS tend to migrate and transport more rapidly to deeper soils and groundwater compared to the longer-chain compounds (≥C7) that are typically retained in shallower surface soils, particularly at the AWI (Chen and others, 2012; Brusseau and others, 2020; Lyu and others, 2022). To better understand the impact of PFAS properties on their transport in subsurface environments, the relationship between partitioning coefficients can be evaluated; for instance, Kd values for long-chain PFAS increase with increasing chain length, signifying the key role of hydrophobicity for PFAS sorption (Guelfo and Higgins, 2013; Lyu and others, 2022). Field sediments and laboratory soils studies have observed strong linear correlations between organic carbon normalized distribution coefficient (log Koc) and chain length for C8–C12 and C7–C10 PFCAs, respectively. Concentrations of PFAS in soil are typically significantly higher than the underlying groundwater. Parts per million concentrations have been measured in soil from PFAS-contaminated sites and are generally orders-of-magnitude greater than background concentrations and typical groundwater concentrations (Brusseau and others, 2020). Depth profiles generally reveal high concentrations at shallow depths with exponential decreases at greater depths, indicating a substantial retention of PFAS in the vadose zone may be occurring during decadal timeframes (Brusseau and others, 2021).
Surface Water
Surface waters can be contaminated with PFAS from runoff from major manufacturing industries synthesizing PFAS, landfill leachates, WWTP discharge, atmospheric deposition, and releases of AFFF at fire training areas, airports, and military installations. In aquatic environments, PFOS and PFOA are expected to be stable to hydrolysis in the environment based on experimentally derived half-lives of 41 and 92 years, respectively (ATSDR, 2021). Similar to the driving interfacial mechanisms in sediment, in water the amphipathic properties of PFAS create a surfactant behavior resulting in the potential for high accumulation at the AWI, specifically the water surface microlayer (Casas and others, 2020; Brusseau and others, 2021; Lyu and others, 2022; Schaefer and others, 2022). In the Southern Ocean, sea-spray aerosols were enriched with PFAS that were two to four orders of magnitude higher than concentrations in bulk seawater and the sea-surface microlayer (Casas and others, 2020). This amplification in sea-spray aerosols was hypothesized to be because of the large surface area of the marine aerosols, the sorption of PFAS to organic matter in the sea-spray, or large amounts of surfactant-like organic matter containing PFAS from the sea-surface microlayer being engulfed in the aerosols.
Vertical profiles of PFAS in the water column may change based on the compound’s particulate and dissolved phase as well as the amount of suspended particles and organic content within the water column; for instance, PFAS profiles were similar between surface and bottom water collected in the South China Sea; however, the concentrations in the surface water were significantly higher than bottom water (Wang and others, 2019). In another field study, no differences were observed in the total or individual concentrations of PFAS in the dissolved phase between the surface, middle, and bottom layers of the water column (Chen and others, 2019). Yet, total concentrations of PFAS in suspended particles were significantly higher in the surface layer particles potentially because of higher surface-area-to-volume ratios of particles and organic content in the upper layer. Short-chain PFAS tend to be more water soluble and are therefore more abundant in water compared to long-chain compounds that tend to bind to particles and accumulate in sediments (Higgins and Luthy, 2006; Chen and others, 2012). Chen and others (2019) determined the perfluoroalkyl chain length was the primary factor affecting the vertical profile distributions in bulk water. Bulk water samples collected in an urban catchment area were dominated by short-chain PFCAs (less than [<] C11) and PFSAs (<C10; Chen and others, 2019). The presence of foam with natural organics was found to enhance PFAS uptake at the water surface (Schwichtenberg and others, 2020; Schaefer and others, 2022); however, the presence of an organic-rich microlayer combined with natural organics enhanced the accumulation of less surface-active compounds (for instance, PFOA and 6:2 FTS) in the surface microlayer whilst inhibiting PFOS accumulation (Schaefer and others, 2022).
Biota
Once in the environment, some PFAS have the propensity to accumulate in biota and some may be enriched in higher trophic levels throughout the food web (in other words biomagnification). Lower PFAS concentrations are typically observed in organisms at the base of the food chain and in lower trophic level invertebrates and fish whereas higher concentrations are more likely to be observed in predatory fish and apex predators (Houde and others, 2011b; Burkhard, 2021; Chen and others, 2021b; ITRC, 2022c; Munoz and others, 2022). The main exposure pathways for biota and animals include contaminated water, diet, and sediment. Although, the mechanisms of PFAS toxicity have not been fully elucidated, they have been associated with a wide range of adverse health effects in humans and animals (ATSDR, 2021). Most of the available human and animal toxicity studies have been on PFOS and PFOA. These studies have demonstrated associations with several cancers, tumors, immunological, developmental, endocrine, reproductive, hepatic system, hematological, neurobehavior, and cardiovascular effects (ATSDR, 2021). Recent studies suggest short-chain PFAS may be equally or in some cases more persistent and toxic than their long-chain counterparts in humans and wildlife, as well as share mechanistic toxicity pathways (Ochoa-Herrera and others, 2016; Nian and others, 2020; Gebreab and others, 2022; Palazzolo and others, 2022; Rericha and others, 2022). Tables 3 and 4 summarize examples of available acute and chronic effects in freshwater and terrestrial biota.
Table 3.
Acute and chronic effects in freshwater taxa.[Modified from Interstate Technology and Regulatory Council (2021a). Refer to table 1 for definitions of the constituents (PFOS, PFOA, PFBS, PFNA, PFBA, PFDA, PFDoA, and PFHxA). mg/L, milligram per liter; >, greater than; d, day; LC50, 50-percent lethal concentration; --, no data available; EC50, 50-percent effect concentration; NOEC, no observed effect concentration; hr, hour; µg/L; microgram per liter]
Taxa | Test organism | Acute effect | Acute effect concentration (95-percent confidence interval) | Chronic effect | Chronic effect concentration | References |
---|---|---|---|---|---|---|
Fish | Zebrafish (Danio rerio) | Developmental/mortality | 6-d EC50: 1.5 mg/L; 6-d LC50 (postfertilization) >10 mg/L | -- | -- | Ulhaq and others, 2013 |
Plant | Duckweed (Lemna gibba) | Frond number, biomass | 7-d EC50: 31.1–59.1 (22.2–60.3) mg/L | -- | -- | Boudreau and others, 2003; ITRC, 2021a |
7-d NOEC: 6.6–29.2 mg/L | ||||||
Invertebrate | Water flea (Daphnia magna) | Survival, immobility | 48-hr EC50: 67.2–130 (31.3–136) mg/L | Survival; reproduction | 5–10 mg/L 21-d NOEC; 9.1 mg/L LC50 | Boudreau and others, 2003; ITRC, 2021a |
48-hr NOEC: 0.8–33.1 (0.6–34.1) mg/L | ||||||
Invertebrate | Water flea (Daphnia pulicaria) | Survival, immobility | 48-hr EC50: 134–169 (103–213) mg/L | -- | -- | Boudreau and others, 2003 |
48-hr NOEC: 13.6–46.9 (2.2–65.3) mg/L | ||||||
Invertebrate | Common blue damselfly (Enallagma cyathigerum) | Developmental, survival | -- | Larval survival; developmental time; metamorphosis success | Long-term NOEC ≤0.01 mg/L | Bots and others, 2010 |
Fish | Fathead minnow (Pimephales promelas) | Survival | 96-hr EC50: 9.1 (7.7–11) mg/L | -- | -- | ITRC, 2021a |
96-hr NOEC: 3.2 mg/L | ||||||
Fish | Rainbow trout (Oncorhynchus mykiss) | Survival | 96-hr EC50: 7.8–22 (6.2–27) mg/L | -- | -- | ITRC, 2021a |
96-hr NOEC: 6.3 mg/L | ||||||
Invertebrate | Mayfly (Neocloeon triangulifer) | Mortality | 96-hr LC50: 82.0 (68.6–90.0) µg/L | Survival | 14-d EC20: 0.804 (0.418–2.057) µg/L | Soucek and others, 2023 |
Crustacean | Common ball water flea (Chydorus sphaericus) | Immobility | 48-hr EC50: 131 mg/L | -- | -- | Ding and others, 2012 |
Fish | Zebrafish (Danio rerio) | Developmental/mortality | 6-d EC50: 350–430 mg/L | -- | -- | Ulhaq and others, 2013 |
Crustacean | Water flea (Daphnia magna) | Immobility | 48-hr EC50: 212–476.52 mg/L | Survival; delayed reproduction | 3.125–6.25 mg/L 7-d NOEC; 6.25–12.5 mg/L 7-d LOEC 1–>100 mg/L 21-d NOEC | Ji and others, 2008; Ding and others, 2012; Barmentlo and others, 2015; ITRC, 2021a |
Crustacean | Water flea (Moina macrocopa) | Immobility | 48-hr EC50: 199.51 mg/L | Fecundity | 3.125 7-d NOEC; 6.25 mg/L 7-d LOEC | Ji and others, 2008 |
Invertebrate | Mayfly (Neocloeon triangulifer) | Mortality | 96-hr LC50 13,451 (11,862–15,254) µg/L | Survival | 14-d EC20: >3085 µg/L | Soucek and others, 2023 |
Crustacean | Water flea (Daphnia magna) | Immobility | 48-hr EC50: 2,183 mg/L | Reproduction; growth (length) | 502 mg/L 21-d NOEC; 995 mg/L 21-d LOEC | Ding and others, 2012; ITRC, 2021a |
Fish | Zebrafish (Danio rerio) | Developmental/mortality | 6-d EC50: 450 mg/L | Growth: development | 4.8 mg/L 7-d LOEC | Ulhaq and others, 2013; Sant and others, 2019 |
6-d LC50 (postfertilization): 1,500 mg/L | ||||||
Fish | Zebrafish (Danio rerio) | Mortality | 72-hr LC50: 84 mg/L | Growth (length and weight) | <0.01–0.1 mg/L 180-d NOEC; 0.01–1 mg/L 180-d LOEC | Zhang and others, 2012 |
Crustacean | Water flea (Daphnia magna) | Immobility | 48-hr EC50: 151 mg/L | Survival; growth (length) | 0.008–0.04 mg/L 21-d LOEC | Ding and others, 2012; Lu and others, 2015 |
Crustacean | Common ball water flea (Chydorus sphaericus) | Immobility | 48-hr EC50: 37 mg/L | -- | -- | Ding and others, 2012 |
Fish | Zebrafish (Danio rerio) | Developmental/mortality | 6-d EC50: 16 mg/L; 6-d LC50 (postfertilization): >10 mg/L | -- | -- | Ulhaq and others, 2013 |
Crustacean | Common ball water flea (Chydorus sphaericus) | Immobility | 48-hr EC50: 462 mg/L | -- | -- | Ding and others, 2012 |
Crustacean | Water flea (Daphnia magna) | Immobility | 48-hr EC50: 185–5,251 mg/L | -- | -- | Ding and others, 2012; Barmentlo and others, 2015 |
Fish | Zebrafish (Danio rerio) | Developmental/mortality | 6-d EC50: 2,200 mg/L | -- | -- | Ulhaq and others, 2013 |
6-d LC50 (postfertilization): > 3,000 mg/L | ||||||
Crustacean | Common ball water flea (Chydorus sphaericus) | Immobility | 48-hr EC50: 26 mg/L | -- | -- | Ding and others, 2012 |
Fish | Zebrafish (Danio rerio) | Developmental/mortality | 6-d EC50: 5 mg/L; 6-d LC50 (postfertilization): 8.4 mg/L | -- | -- | Ulhaq and others, 2013 |
Crustacean | Water flea (Daphnia magna) | Immobility | 48-hr EC50: 163 mg/L | -- | -- | Ding and others, 2012 |
Crustacean | Water flea (Daphnia magna) | Immobility | 48-hr EC50: 66.3 mg/L | -- | -- | Ding and others, 2012 |
Crustacean | Common ball water flea (Chydorus sphaericus) | Immobility | 48-hr EC50 28 mg/L | -- | -- | Ding and others, 2012 |
Crustacean | Common ball water flea (Chydorus sphaericus) | Immobility | 48-hr EC50: 19 mg/L | -- | -- | Ding and others, 2012 |
Crustacean | Water flea (Daphnia magna) | Immobility | 48-hr EC50: 133 mg/L | -- | -- | Ding and others, 2012 |
Crustacean | Water flea (Daphnia magna) | Immobility | 48-hr EC50: 1,048 mg/L | Population growth rate | 853 mg/L 21-d EC50 | Barmentlo and others, 2015 |
Table 4.
Acute and chronic effects in terrestrial taxa.[Modified from Interstate Technology and Regulatory Council (2021a). Refer to table 1 for definitions of the constituents (PFOS, PFOA, PFBS, PFHxS, PFNA, and PFHpA). EC50, 50-percent effect concentration; >, greater than; mg/kg, milligram per kilogram; NOAEC, no observed adverse effect concentration; <, less than; --: no data; LOAEC, lowest observed adverse effect concentration; LD50, 50-percent lethal dose; LC50, 50-percent lethal concentration; EC10, 10-percent effect concentration; L, liter; LOAEL, Lowest Observed Adverse Effect Level; bw/d, body weight per day; mg/kg/bw/d, milligram per kilogram per body weight per day; mg/kg/d, milligram per kilogram per day; sig. diff, significant difference; LOEC, lowest observed effect concentration]
Taxa | Test organism | Acute effect | Acute effect concentration | Chronic effects | Chronic effect concentration | References |
---|---|---|---|---|---|---|
Plant | Various crop plants (onion, etc.) | Survival, emergence, shoot height/weight | 21-day EC50: 57– >1,000 mg/kg soil; | -- | -- | Beach and others, 2006; ITRC, 2021a |
21-day NOAEC: <3.91–1,000 mg/kg soil | ||||||
Invertebrate | Red wiggler worm (Eisenia fetida) | Survival, mortality | 14-day NOAEC: 77 mg/kg soil | Mortality; survival; growth | 30-day NOEC: 1 mg/kg soil; 42-day NOEC: 80 mg/kg soil; LOEC: 120 mg/kg; 28-day LC50: 447 mg/kg soil | Beach and others, 2006; Xu and others, 2013; Zhao and others, 2014; ITRC, 2021a |
14-day LOAEC: 141 mg/kg soil | ||||||
14-day LD50: 373 mg/kg soil | ||||||
Plant | Common wheat (Triticum aestivum) | Growth | 7-day NOEC: 100 mg/kg soil | Mortality; growth | 30-day NOEC: 1 mg/kg soil | Qu and others, 2010; Zhao and others, 2014 |
Plant | Lettuce (Lactuca sativa) | Growth | 5-day EC10: 24 mg/kg soil | -- | -- | Li, 2009 |
Plant | Cucumber (Cucumis sativus) | Growth | 5-day NOEC: >200 mg/kg soil | -- | -- | Li, 2009 |
Plant | Bok choy (Brassica rapa chinensis) | Growth | 5-day EC10: 71 mg/kg soil; 7-day EC10: 72 mg/kg soil; EC50 ranged from 95–>200 mg/kg | -- | -- | Li, 2009; Zhao and others, 2011 |
Invertebrate | Grey earthworm (Aporrectodea caliginosa) | -- | -- | Survival | 40-day NOEC: 1 mg/kg soil | Zareitalabad and others, 2013 |
Microorganism | Soil bacteria | -- | -- | Biomass | 40-day NOEC: 500 mg/kg soil | Zareitalabad and others, 2013 |
Invertebrate | Buff-tailed bumblebee (Bombus terrestris) | -- | -- | Mortality | LC50 of 1.01 mg PFOS/L sugar water | Mommaerts and others, 2011 |
Invertebrate | Soil mite (Oppia nitens) | -- | -- | Reproduction | 23 mg/kg (sandy loam)–95 mg/kg (clay loam) | Princz and others, 2018 |
Invertebrate | Springtails (Folsomia candida) | -- | -- | Reproduction | 94 mg/kg (sandy loam)–233 mg/kg (clay loam) | Princz and others, 2018 |
Vertebrate—Avian | Northern bobwhite (Colinus virginianus) | -- | -- | Successful hatching | 90-day parental exposure: 0.00245mg/kg (bw/d) | Dennis and others, 2020 |
Body weight | 140-days: LOAEL 0.77 mg/kg/bw/d) | Newsted and others, 2005 | ||||
Vertebrate—Avian | Japanese quail (Coturnix japonica) | Mortality (ADD50) | 5-day, treated feed: 38–130 mg/kg/d (LC50: 351–467 mg/kg) | -- | -- | Bursian and others, 2021 |
Vertebrate—Avian | Japanese quail (Coturnix japonica) | Mortality (ADD50) | 5-day, treated feed: 55 mg/kg/d (LC50: 398 mg/kg) | -- | -- | Bursian and others, 2021 |
Vertebrate—Avian | Japanese quail (Coturnix japonica) | Mortality (ADD50) | 5-day, treated feed: 68 mg/kg/d (LC50: 496 mg/kg) | -- | -- | Bursian and others, 2021 |
Plant | Common wheat (Triticum aestivum) | NA | NA | Growth | 30-day NOEC: 1 mg/kg | (Zhao and others, 2014) |
Plant | Lettuce (Lactuca sativa) | Growth | 5-day EC50: 170 mg/kg | -- | -- | Li, 2009 |
Plant | Cucumber (Cucumis sativus) | Growth | 5-day NOEC: 812 mg/kg | -- | -- | Li, 2009 |
Plant | Bok choy (Brassica rapa chinensis) | Growth | 5-day EC10: 155 mg/kg soil; 7-day EC10: 103 mg/kg soil; EC50 ranged from 107 to 246 mg/kg | -- | -- | Li, 2009; Zhao and others, 2011 |
Microorganism | Soil bacteria | -- | -- | Biomass | 40-day NOEC: 500 mg/kg soil | Zareitalabad and others, 2013 |
Microorganism | Soil bacteria | -- | -- | Enzyme activity | 28-day EC50: 62.35–93.31 mg/kg soil | He and others, 2016 |
Invertebrate | Grey earthworm (Aporrectodea caliginosa) | -- | -- | Survival | 40-day LC50: 84 mg/kg soil | Zareitalabad and others, 2013 |
Invertebrate | Red wiggler worm (Eisenia fetida) | -- | -- | Growth | 28-day NOEC: 10 mg/kg soil; LOEC: 25 mg/kg | He and others, 2016 |
Invertebrate | Red wiggler worm (Eisenia fetida) | Mortality | 1 mg/kg | Growth (weight loss) | 21-day, sig. diff. observed at 100 mg/kg although no NOEC/LOEC estimated. | Karnjanapiboonwong and others, 2018 |
Invertebrate | Red wiggler worm (Eisenia fetida) | Mortality | 100 mg/kg | Growth (weight loss) | 21-day, sig. diff. observed at 100 mg/kg although no NOEC/LOEC estimated. | Karnjanapiboonwong and others, 2018 |
Invertebrate | Red wiggler worm (Eisenia fetida) | Mortality | 100 mg/kg | Growth (weight loss) | 21-day, sig. diff. observed at 100 mg/kg although no NOEC/LOEC estimated. | Karnjanapiboonwong and others, 2018 |
Invertebrate | Red wiggler worm (Eisenia fetida) | Mortality | 100 mg/kg | Growth (weight loss) | 21-day, sig. diff. observed at 100 mg/kg although no NOEC/LOEC estimated. | Karnjanapiboonwong and others, 2018 |
In living organisms, PFAS bind to the protein albumin in blood, liver, and eggs (ATSDR, 2021). Substantial bioaccumulation and biomagnification is more frequently observed among the long-chain PFAS (Ahrens and Bundschuh, 2014; Chen and others, 2021a; Chen and others, 2021b). Evidence suggests these behaviors are directly related to the carbon chain length, where the bioaccumulation potential increases with increasing chain length (≤C10), then the bioaccumulation potential decreases with chain lengths greater than C10 (≥C10). In animals the bioaccumulation potential of PFSA is higher compared to PFCA (Conder and others, 2008); for example, PFOS (a PFSA) typically dominates concentration profiles in wildlife including apex predators worldwide, whereas PFOA (a PFCA) only represents a minor (< 2 percent) constituent (Houde and others, 2011a; Chen and others, 2021b). Long-chain PFCAs (C9–14) were also found to be substantial in apex predators with higher proportions among the odd-chain length PFCAs compared to the even-chain length compounds; nonetheless, short-chain PFAS and their metabolites should not be overlooked since increasing evidence suggests their accumulation patterns may be similar to long-chain compounds (Nilsson and others, 2013; Kabadi and others, 2018; Rice and others, 2020). Some studies have found short-chain PFAS have strong binding affinities and may be retained in blood longer than the long-chain PFAS (Chen and others, 2021b; Li and others, 2021).
Potential PFAS exposure sources in plants may include contaminated water, soils, land application of biosolids, pesticide applications, landfill leachates, PFAS releases at usage areas (for instance, fire training areas), industrial emissions, and atmospheric deposition (Adu and others, 2023). Accumulation of PFAS in plants (including trees, fruits, and agricultural crops) occurs primarily through root uptake as well as translocation, active transport, and passive diffusion (Wang and others, 2020; Adu and others, 2023). Studies have demonstrated correlations between soil clay contents and PFAS concentrations in porewater, which governs PFAS bioavailability to plants (Adu and others, 2023). Because of the proteinophilic nature of PFAS, the accumulation of PFOS and PFOA in roots were positively correlated with root protein contents. This suggests the importance of protein as a translocation pathway, which was further demonstrated by significant correlations of translocation factors with the ratios between shoot and root protein content (Wen and others, 2016). The long-chain PFAS preferentially accumulate in the roots with higher accumulation of the more mobile short-chain PFAS in the aboveground shoots of the plants (Felizeter and others, 2012, 2014; Krippner and others, 2014; Krippner and others, 2015; Wang and others, 2020). Increased uptake and transport from the roots to the shoots may be amplified by increased salinity and temperature (Zhao and others, 2016). The main biotic factors that may influence the uptake of emerging chemicals of concern (including PFAS) in crop plants include the plant variety, genotype, and physiology as well as the composition of soil microorganism communities (Christou and others, 2019). The abiotic factors influencing chemical uptake in plants include the climatic and environmental conditions and perturbations (Christou and others, 2019). In contrast to animals, there is some indication that PFCA may bioaccumulate to a higher degree in plants compared to PFSA (Blaine and others, 2014; Krippner and others, 2015). Ultimately the fate and transport of PFAS in the environment is influenced by the species physiology, abiotic factors, and the physicochemical properties of the environmental media and PFAS compounds.
3. Baseline Considerations
The simple definition of baseline data are data collected and measured before the start of a project or before an environmental impact for later comparison. For Natural Resource Damage Assessment and Restoration (NRDAR), baseline is defined as “the condition or conditions of a natural resource that would have existed at the assessment area had the discharge of oil or release of the hazardous substance under investigation not occurred” (Bureau of Land Management, 2008, p. 3; 15 CFR §990.30, p. 407). In NRDAR, baseline may be established using pre-release data or by using a control or reference area(s).
Although PFAS have been in use for about 70 years, only recently (~10–20 years) have they been measured routinely in environmental media primarily because these chemicals have not been regulated, reliable analytical methodology may not have been available, and the contamination extent and associated health effects were largely unknown. Presently, PFAS have been detected globally in all environmental media and all regions of the globe. Since PFAS are synthetic chemicals, a “natural background” concentration does not exist in environmental media; therefore, several states and institutions attempt to establish an “anthropogenic background” concentration in environmental media (Ahrens and others, 2016; Brusseau and others, 2020; Zhu and others, 2022). Knowledge of the surrounding area and the identification of potential industrial sources will help identify potential direct sources; however, if there are no potential direct industrial sources within the surrounding area, it is important to consider other anthropogenic sources of PFAS (for instance, atmospheric deposition, biosolid applications) when establishing baseline. As a reminder for NRDAR practitioners, at least one viable potentially responsible party needs to be identified before initiating a CERCLA NRDAR. If aerial emissions are considered a potential pathway of contamination, additional source apportionment and analyses may be required to establish liability.
Approaches for sites without a known source may start with a comprehensive preliminary assessment to further understanding of potential PFAS usage in the area, history of site operations and chemical usage, and the regional geologic and hydrologic framework as it relates to contaminant transport in the environment (ITRC, 2023). Comparing the site timeline with the timeline of PFAS development and use in the region can help identify potential sources or releases; for example, a historical timeline could be useful if the site or surrounding area was used for fire training activities, a landfill, biosolid application, or if there is a nearby airport, military installation, or WWTP. Characterizing and comparing PFAS compositional profiles in multiple environmental media to available data from nearby source(s) may help to associate or dissociate the contamination with a particular source (sec. 9). It also is crucial to understand PFAS precursors and degradation products when evaluating exposure scenarios and source identification, particularly if the site evaluation includes historical contamination. Precursors released into the environment may degrade and transform into PFAA (such as PFOA and PFOS) and should be considered in risk assessments (Glaser and others, 2021; Pickard and others, 2022). Site characterization can also be supported by incorporating models to predict air mass trajectories (for example HYSPLIT or CMAQ), groundwater plumes and subsurface flow (for example 3DFATMIC, Bioplume III), or exposure models in soil and the vadose zone (for example EMSOFT, PRZM). When identifying PFAS sources, important factors to consider include data quality, target PFAS lists, precursor and degradation products, temporal effects, spatial effects, proper sampling techniques, and weight of evidence (ITRC, 2023). Examples of perfluoroalkyl carboxylic acid (PFCA) precursors include the following:
-
● Perfluoroalkanoyl fluorides (PFA)
-
● Perfluoroalkyl iodides (PFAI)
-
● Perfluoroalkyl aldehydes (PFAL)
-
● Polyfluoroalkyl ether sulfonic acids (PFESA)
-
● Polyfluoroalkyl ether carboxylic acids (PFECA)
-
● Chloropolyfluoroalkyl ether acids
-
● Chloropolyfluoroalkyl acids
-
● Fluorotelomer Substances (for example, FTSA, FTCA, FTUCA, FTOH)
-
● Perfluoroalkane sulfonyl fluorides (PASF)
-
● Perfluoroalkane sulfonamides (FASA)
-
● Perfluoroalkane sulfonamido substances (for example, MeFASA, EtFASA, BuFASA, FASEs, MeFASE, EtFASE, MeFASAA, EtFASAA)
-
● 8:2 FTOH → 8:2 FTAL → 8:2 FTCA → 8:2 FTUCA → 7:2 sFTOH → PFOA (aerobic biotransformation pathway)
-
● 8:2 FTOH → 8:2 FTAL → 8:2 FTCA → 8:2 FTUCA → 7:3 UAcid → 7:3 Acid, PFPA, PFHxA, PFHpA (aerobic biotransformation pathway)
-
● 8:2 FTOH → 8:2 FTAL → free-radical and transient molecular intermediates → stable molecular intermediates → PFNA, PFHpA, PFHxA, PFPeA, PFBA, PFPrA, TFA (atmospheric degradation)
-
● 8:2 FTOH → 8:2 FTAL → 8:2 FTCA → PFNA
-
● 8:2 FTOH → 8:2 FTAL → 8:2 FTCA → 8:2 FTUCA → molecular intermediates → 7:2 sFTOH → PFOA (metabolic degradation)
-
● 8:2 FTOH → 8:2 FTAL → 8:2 FTCA → 8:2 FTUCA → molecular intermediates → 7:3 FTUCA → PFHpA (metabolic degradation)
-
● 8:2 FTOH → 8:2 FTAL → 8:2 FTUAL → 7:3 FTUAL → 7:3 FTAL → 7:3 FTCA → PFOA, PFHxA, PFPeA, 7:3 FTCA (metabolic degradation)
-
● 10:2 FTOH → 10:2 FTCA → 10:2 FTUCA → PFNA, PFDA, PFHxA
-
● POSF → PFOS (product manufacture)
-
● POSF → FOSA → PFOS (metabolic degradation)
-
● POSF → EtFOSE → EtFOSAA → FOSAA → FOSA → PFOS (metabolic degradation)
-
● POSF → MeFOSE → MeFOSAA → FOSAA → FOSA → PFOS (metabolic degradation)
-
● 6:2 and 8:2 FTSA → 5:3 and 7:3 FTCA → 3:3 FTCA (major compound of legacy landfill leachates)
-
● Bis(perfluorooctyl)phosphic acid (PFPiA) → molecular intermediates → PFOA (metabolic degradation)
-
● N-EtFOSA → FOSA → FOSI → PFHxS, PFOS, PFBS (metabolic degradation)
-
● FOSA → PFBS, PFHS (metabolic degradation)
-
● FOSA → FOSI → PFOS (metabolic degradation)
4. Response Considerations
When developing a NRDAR, it is important to understand response and remedial actions and how those may contribute to the injury of natural resources. Responders to accidental spills or releases of oil or hazardous materials may need to pay particular attention to the types of gear needed to avoid inadvertently introducing or augmenting PFAS in the environment; for example, a commonly used fire suppressant was used in an emergency response to prevent the ignition of vapors at one of the largest onshore gas spills in the United States in more than 20 years. Questions emerged when elevated concentrations of PFAS were detected at the spill site (Edelstein, 2021; Siros and Lawson, 2021). Emergency spill responders are faced with two challenges: using efficient products to contain spills and fires as well as ensuring that these products do not pose a potential threat to human and environmental health. If fluorinated AFFF foams are a necessity, then containing and removing the foams and runoff as quickly as possible could help limit the extent of contamination and potential injury to natural resources because of response actions.
Additionally, natural resource agencies may provide technical assistance to the response to implement hazing techniques to deter wildlife or migratory birds and waterfowl from using areas affected by PFAS releases including PFAS foam and any areas containing PFAS runoff. Implementing hazing could reduce potential NRDAR liability by reducing exposure of additional natural resources to hazardous substances. Additional cleanup information for PFAS contaminated sites, site characterization, interim recommendations for addressing groundwater contamination, and interim Naval Facilities Engineering Systems Command site guidance may be provided by individual states and can also be found at the EPA, the ITRC, and Department of the Navy (ITRC, 2022e; Department of the Navy, 2023; EPA, 2023g, h).
5. Ephemeral Data Considerations During Response
Ephemeral data collection includes early sampling and data collection that is done “before completing the preassessment screen to preserve data and materials that are likely to be lost if not collected at that time and that will be necessary to the natural resource damage assessment” (Bureau of Land Management, 2008, p. 4; 15 CFR §990.43). For oil spills, the trustees can do data collection and analyses (for example, collecting ephemeral data) during the preassessment phase provided it is coordinated with response actions (15 CFR §990.43). Ephemeral data collection should be coordinated closely with the lead response agency so that efforts are not duplicated and so that NRDAR-specific activities are cleared by the unified command (for example clearance for site access and safety). See 43 CFR §11.22 for examples of ephemeral data that may be collected before completing the preassessment screen (15 CFR §990.43). Refer to section 8 for additional suggestions that may also pertain to ephemeral data.
If products containing PFAS (for example AFFF) are anticipated to be involved in response actions, released during an incident, or present because of potentially responsible party activities at a site, the need to collect ephemeral data should be evaluated. A source sample of PFAS-containing foam or product used at the site should be collected by coordinating with the lead response agency and following appropriate sample collection guidelines for the matrix. A detailed guide to AFFF, effects and tradeoffs of using during response activities is available from National Oceanic and Atmospheric Administration (Abt Associates, 2023). Applying AFFF on land may result in the direct release of PFAS on vegetation, soils, or other surfaces that may follow surface-runoff pathways into streams or sewer drains and release into larger water bodies. Once in the soil, PFAS can slowly leach into underlying soils, the vadose zone, and may eventually reach groundwater following the flow path, furthering the extent of contamination. Applying AFFF over water may result in PFAS mixing in the water column exposing aquatic organisms and sediments (sec. 2) for partitioning information). Should the application of AFFF be required, samples that should be collected as soon as possible after the application may include a source sample, surface water samples and surface water foam samples, subsurface water, sediment, or soil samples (Abt Associates, 2023). If additional resources are exposed, then additional samples may be required (for example terrestrial biota, groundwater, aquatic organisms with high site fidelities). As formulations of firefighting foams containing PFAS are phased out, it may still be necessary to verify whether AFFF containing PFAS was previously used at the site. Comparing compositional profiles from the different formulations and sources combined with determining the presence or absence in multiple environmental media may provide additional support and evidence for site evaluations.
6. Restoration Considerations
Similarly, reevaluating standard remedial strategies and restoration efforts may also be warranted. A commonly used technique for metal immobilization of mining-impacted lands is the application of biosolids, biosolid-based composts, or other composts to reduce the bioavailability, mobility, and subsequent biological effects from metals at these sites. In addition, soil amendments provide nutrients—especially nitrogen and phosphorus—that promote the establishment and growth of vegetation, reduce use of fertilizer, and improve soil structure. During a nationwide survey of biosolids in the United States, PFAS were detected in the parts per billion range, with PFOS as the most abundant followed by PFOA (Venkatesan and Halden, 2013). PFAS from a variety of resources may accrue in WWTP sludges, creating secondary release sources in areas where biosolids are produced, applied, and disposed; however, the significance of these releases will depend on the PFAS composition (which is a function of the WWTP influent sources and processes), environmental controls, and release mechanisms (application rates, frequency, and duration; ITRC, 2022b). Higher PFAS concentrations in sewage sludge are associated with industrial wastewater discharges, although exceptions may occur. Approximately 51 percent of the sewage sludge produced in the United States is applied to agricultural land as biosolids (Mills, 2020; ITRC, 2022b). Also, longer-chain PFAS like PFOS and PFOA in biosolids applied to land tend to accumulate in near-surface soils. PFAS concentrations in soil are proportional to application rate and frequency of application (Pepper and others, 2021); therefore, implementors of restoration should consider the risk of the potential addition of PFAS or other contaminants through the use of biosolid amendments. Treatment and removal technologies of PFAS are not sufficient for complete remediation since they do not necessarily remove, degrade, or destroy these chemicals; instead, removal only transfers and concentrates PFAS from one medium to another (Dickman and Aga, 2022). Best management practices, such as the following examples, should be considered when including biosolids in restoration approaches: avoiding application of biosolids on steep slopes and floodplains, using vegetative buffers strips along waterways, following minimum setback distances from sensitive areas, and aging of humification of biosolids to help limit bioavailability of contaminants (Oun and others, 2014). Lastly, be familiar with state-specific guidance and laws on PFAS in biosolids, as several states are in the process of considering and (or) developing regulations for PFAS in biosolids application to land; for example, on April 15, 2022, Maine passed a bill (LD 1911; Maine State Legislature, 2022) banning the use of biosolids containing PFAS in land applications and sale of fertilizer or compost products derived from PFAS-containing biosolids unless it can be determined that the biosolids are PFAS-free.
7. Sampling Techniques and Collection Preparation Considerations
Because of the extensive use of PFAS in the manufacturing and production of countless pieces of field gear and collection containers, sampling procedures require additional considerations when selecting techniques and gear for collecting environmental media for PFAS analysis; for instance, water and stain-resistant clothing (for example Gore-Tex), water-repellant paper (for example Rite in the Rain), and select jar liners (for example Teflon) are coated or made with PFAS materials and are not optimal because of the potential of PFAS materials leaching into the sample.
Any items or materials that will come into direct contact with the sample that have not been verified to be PFAS-free should be screened. There is inconclusive evidence that field and sampling gear may plausibly impact sample PFAS concentrations (Denly and others, 2019; Rodowa and others, 2020); therefore, it is still recommended to avoid materials containing PFAS (for example, Teflon, PTFE, fluoropolymers) and implement a Quality Assurance Project Plan that includes the appropriate use of laboratory (for example method blanks, laboratory control samples, laboratory control sample duplicate, field duplicate, matrix spike, and matrix spike duplicates) and field (for example duplicates, replicates, field blanks, equipment blanks, trip blanks) quality control samples. Safety data sheets and ingredient lists should be reviewed to avoid products containing PFAS. Individual states and contract laboratories that have developed sampling guidelines may offer further recommendations on sampling protocols; thus, it is advised to refer to the proper protocols provided by the state where samples are to be collected or the selected contract laboratory before collection. Examples of common personal care products, clothing, field gear, sampling equipment materials, and miscellaneous supplies that may incorporate PFAS in the manufacturing process include (but not limited to):
-
● Personal care products that may contain PFAS: cosmetics, lotion, moisturizers, sunscreens, bug repellant, shampoo, laundry detergent, fabric softener, and so on.
-
● PFAS-coated (for example, Teflon, PFOA or PTFE) or PFAS-treated clothing, shoes, and textiles for water and stain resistance or repellency.
-
● Field gear that may either contain or have the potential to leach or adsorb PFAS materials include fluoropolymer materials used in bailers, valves tubing, pump bladders, pipe thread seal tape (Teflon tape), low-density polyethylene (LDPE) materials (Hydrasleeves or Ziploc brand bags).
-
● PFAS equipment materials: polytetrafluroethylene (PTFE), polyvinylidene fluoride (PVDF), polychlorotrifluoroethylene (PCTFE), ethylene-tetrafluoroethylene (ETFE), fluorinated ethylene propylene (FEP), Teflon, Hostaflon, Kynar, Neoflon, Fefzel.
-
● Miscellaneous supplies that may contain PFAS: Post-it notes, blue ice, plastic clipboards, waterproof paper or field books/logs, felt-tip markers, Decon 90 laboratory detergent, nonstick aluminum foil.
Examples of PFAS-free options for common personal care products, clothing, field gear, sampling equipment materials, and miscellaneous supplies include the following (but not limited to):
-
● PFAS-free personal care products: where possible avoid wearing cosmetics, lotions, shampoos, or moisturizers while sampling unless the ingredients can be verified PFAS-free. PFAS-free bug repellents include those that use 100-percent natural ingredients or DEET (for example OFF Deep Woods and Sawyer Permethrin). PFAS-free sunscreens include those that use 100-percent natural ingredients or mineral-based (for example zinc oxides and titanium).
-
● PFAS-free clothing, shoes, and textiles: Clothing made of synthetic or natural fibers, neoprene, polyvinyl chloride, wax-coated fabrics (for example steel toed boots made with polyurethane and polyvinyl chloride).
-
● PFAS-free field gear: select gear that contain high-density polyethylene, silicone, or polypropylene materials.
-
● PFAS-free miscellaneous supplies: bagged ice (nonchemical), aluminum clipboard, untreated paper and field books, ball point pens, pencils, Alconox or Liquinox laboratory detergent, dull-side of nonstick aluminum foil.
PFAS have been used in countless consumer products, including laboratory supplies and scientific equipment; thus, the material of collection containers and equipment is an important consideration to reduce sample contamination or concentration losses. There is some indication that there may be sorption losses of PFAS analytes on certain materials or artificial increases in concentrations because of leaching from materials containing PFAS. Lath and others (2019) examined sorption losses of PFOA on a variety of glass and plastic centrifuge tubes and syringe filters. Data from this study demonstrated greater sorption losses of PFOA on polypropylene containers compared to glass containers. In addition, a study done by the EPA recently identified eight common PFAS compounds have the potential to leach from the walls of fluorinated high-density polyethylene containers into the samples contained (Nguyen, 2022). Denly and others (2019) tested different materials to evaluate possible worst-case scenarios after allowing leaching to occur in bottled water during a 24-hour period. Leachates from low-density polyethylene and PTFE tubing only had detectable concentrations of PFCA. The low-density polyethylene tubing contained the highest concentrations, with PFBA and PFPeA dominating profiles at similar concentrations (mean of ~600 nanograms per liter [ng/L]). Individual PFSA and PFCA were detected at low parts per trillion concentrations (<100 ng/L) in the leachate from other materials (bailer line, sample labels, nitrile gloves, and field book covers). No PFAS were detected in the leachate of aluminum foil, resealable plastic storage bags, passive diffusion bag, polyvinyl chloride pipe, silicone tubing, polyethylene bladder among a few other materials; however, it is important to note that adsorption or leaching processes of PFAS may differ widely under different conditions (for instance solution chemistry, matrix, holding times, and temperatures).
Container selection may depend on the matrix and analytical method (table 5); however, the widely accepted methods typically require either polypropylene or high-density polyethylene containers. Analytical methods often include recommendations to prepare for sample collection, such as rinsing and testing containers. Additionally, contract laboratories may supply PFAS-free containers along with PFAS-free reagent water that have been tested and confirmed for use for environmental and field quality-control (QC) samples. Field QC samples are critical for monitoring background interference and concentrations. Typically, QC samples include equipment blanks, field reagent blanks, duplicates, method blanks, project-specific matrix spikes, spiked duplicates, and standard or certified reference materials; therefore, before any field collections, it is advised to generate a project Quality Assurance Project Plan and consult the laboratory doing the analysis and the selected method for recommendations and requirements. A data management plan should be used to outline the plan to acquire, process/analyze, preserve, and publish/share study data. Federal agency practitioners are encouraged to follow their agency’s guidance for meeting the requirements of the 2018 Geospatial Data Act (43 U.S.C. 2801 et. seq.), 2018 OPEN Government Data Act (Public Law 115–435, 44 U.S.C. 101 note), and the Foundations for Evidence-Based Policymaking Act of 2018 (Public Law 115–435) in preparing data and metadata.
Table 5.
Commercially available method details for per- and polyfluoroalkyl substances analysis.[EPA, U.S. Environmental Protection Agency; SW, solid waste; ASTM, American Society for Testing and Materials; MDL, method detection limit; pptr, part per trillion; g, gram; mL, milliliter; HDPE, high-density polyethylene; g/L, gram per liter; °C, degree Celsius; ≤, greater than or equal to; d.w., dry weight; w.w., wet weight]
Other sampling techniques may include passive sampling devices (PSDs), which have been used for decades for monitoring hydrophobic and hydrophilic organic compounds in air and aquatic environments (Alvarez, 2010). The first reported use of PSDs for monitoring PFAS was using the Polar Organic Chemical Integrative Sampler (POCIS) for monitoring wastewater treatment plant effluent (Alvarez and others, 2007). Optimization of methods and determination of uptake kinetic variables for measuring select PFAS in POCIS was first published in 2010 (Erhunse, 2010). Since then, experimental studies have evaluated a variety of PSDs for monitoring PFAS in groundwater, porewater, and surface water (Alvarez, 2010; Gong and others, 2018; Imbrigiotta and Harte, 2020; Taylor and others, 2021; Pronschinske and others, 2023). The more commonly used and commercially available PSDs for sampling PFAS in surface water include POCIS and the PFASsive, whereas the DMPDB and HydraSleeve are the more common and commercially available PSDs for sampling PFAS in groundwater. The advantages of using PSDs include ease of use, reduced field time, no depth limitations, and their adaptability for long-term monitoring. Disadvantages may include sample volume restrictions, deployment time requirements, extraction and calibration requirements, and chemical constituent limitations.
8. Sampling Design Considerations
Sampling design considerations will be determined based on the site-specific details including the assessment area, potential extent of contamination, and specific research questions. Understanding the physicochemical properties of PFAS and the environmental media can help direct research questions and assist with the selection of appropriate environmental matrices, biota, and target organs or tissues (sec. 2). Concentrations and composition characteristics of PFAS in environmental media (for instance air, sediments, water, fish) can fluctuate considerably among seasons, location, and species (Salice and Suski, 2022; Wang and others, 2022). Precipitation, drought, and the hydrodynamics of a system can drastically alter concentrations in surface water and biota. These changes may be highly dependent on the hydrodynamics of a system, trophic level, and species-specific differences in uptake, elimination, and metabolism. For areas near WWTPs, discharge variations may also cause large variations in surface water concentrations (Bowman, 2019).
In NRDAR, natural resources are defined as “land, fish, wildlife, biota, air, water, ground water, drinking water supplies, and other such resources...” (15 CFR § 990.30, 1986, p. 409). Natural resource services are defined as “the physical and biological functions performed by the resource including the human uses of those functions. These services are the result of the physical, chemical, or biological quality of the resource” (U.S. Department of the Interior, 2023; 15 CFR §990.30). Natural resource services can include services to other resources (such as providing food, shelter, or nesting habitat) or directly benefiting humans or human use (for example fishing and recreational activities). Selecting matrices with available toxicity data or toxicity thresholds can help inform meaningful injury assessments. Species-specific toxicity data may be limited, but the Agency for Toxic Substances and Disease Registry and the ITRC have compiled toxicological profiles and available effect concentrations for humans and wildlife (ATSDR, 2021; ITRC, 2021a). Section 1 of this report briefly discusses environmental fate and transport and provides some examples of acute and chronic effects for select freshwater and terrestrial taxa (tables 3 and 4).
Persistent organic pollutants that bioaccumulate or biomagnify, such as dichlorodiphenyltrichloroethane (better known as DDT) and polychlorinated biphenyls (PCB), are considered hydrophobic and lipophilic, which sequester in lipid rich tissues. In contrast, PFASs are considered amphipathic (both hydrophobic and hydrophilic) and proteinophilic compounds that preferentially bind to proteins and concentrate primarily in blood and liver (Martin and others, 2003a; Taniyasu and others, 2003; Conder and others, 2008; Ahrens and others, 2009; Shi and others, 2012; Ng and Hungerbuehler, 2013; Ng and Hungerbühler, 2014). Supporting research using targeted analysis has demonstrated that water concentrations and PFAS profiles are not a good proxy for estimating fish concentrations; therefore, it is recommended to include measuring concentrations in fish (Lam and others, 2017; Pickard and others, 2022) and potentially other aquatic biota. Studies have demonstrated the tissue distribution of PFAS in fishes accumulated highest in blood or plasma followed by liver or kidney and the lowest concentrations are typically in the adipose tissue and muscle (Martin and others, 2003b; Bangma and others, 2017b). Yet tissue selection and required volumes and mass may be dependent on the research question and analytical method, respectively; for example, if the goal is to assess human dietary risks, then using community and population knowledge to sample relevant edible portions (muscle or organs) of the organisms of interest would be necessary. This could include plants and whole-body assessments of wild game and fish. Additional tissues or matrices, such as blood or liver, may be required to assess exposures and specific health-related endpoints in human populations and wildlife; furthermore, if the focus is on a food web study, then whole organism sampling may be necessary.
9. Analytical Methods
PFAS analytical methods differ based on regulatory requirements and matrix type. Tables 5 and 6 summarize the analytical method details for different matrices and the different analytes included in each method. These tables include method information, collection container requirements, holding times, preservation requirements, analytical instrumentation, analytes included, detection limits, and more. More information regarding method requirements and method comparisons can be found from the EPA, ASTM and ITRC (ASTM, 2021; ITRC, 2022a; EPA, 2023i). The main differences among the available methods are mainly the validated matrix (for example drinking water vs nonpotable), sample cleanup techniques, the number of target analytes, and the instrument limits of detection. To help guide the selection of a particular method, this section provides a brief description of commonly accepted methods for different media and matrices.
Table 6.
List of analytes included in commercially available methods for analyzing per- and polyfluoroalkyl substances (PFAS).[Refer to table 1 for definitions of the analytes. CASRN, Chemical Abstracts Service Registry Number; EPA, U.S. Environmental Protection Agency; SW; solid waste; ASTM; American Society for Testing and Materials; X, analyte included in method; --, not included in method; NA, not applicable]
Drinking Water
A study comparing three commonly used methods for drinking water (EPA 537, ASTM D7979, and ISO CD21675) demonstrated similar accuracy and precision, method reporting limits, and quality assurance and QC acceptance protocols among these methods (Lipps, 2018). Regardless, PFAS environmental investigations should be done in compliance with applicable regulatory requirements (for example Safe Drinking Water Act, 42 U.S.C. §300f et seq.; U.S. Department of Defense [DoD]) that may differ with State, regional, or Provincial requirements (ASTM, 2021). The analysis of public water supplies in the United States is regulated under the Safe Drinking Water Act and will likely require one of the two validated methods for this matrix (either EPA Method 537.1 or 533), which mainly differ in the number of target analytes and the quantitation method (EPA, 2019; Shoemaker and Tettenhorst, 2020). A list of EPA approved laboratories that have met the proficiency testing for these two methods under the fifth Unregulated Contaminant Monitoring Rule is updated regularly on the EPA website (EPA, 2022a). The International Organization for Standardization (ISO) also provides analytical methods for analyzing unfiltered drinking water and natural water (ISO Methods 21675 and 25101).
Nonpotable Water, Environmental Matrices, Biological Tissues, and Fluids
Acceptable methods for nonpotable water, environmental matrices, and biological tissues and fluids include the following:
-
● EPA Method 1633 (aqueous, solid, biosolid, and tissue matrices)
-
● EPA Method 3512 (solvent dilution of nonpotable waters)
-
● EPA Method 8327 (large volume inject for aqueous and other matrices)
-
● ASTM Methods D7968 (large volume injection for soil, validated for 21 PFAS)
-
● ASTM D7969 (large volume inject for water, validated for 21 PFAS)
-
● ASTM D8421 (large volume inject for aqueous matrices; validated for 44 PFAS)
-
● DoD AFFF01 (AFFF formulations)
-
● ISO 25101 (PFOS and PFOA in water)
-
● ISO 21675 (PFAS in water)
-
● FDA CAM Method (food)
-
● Centers for Disease Control 6304 (blood/serum).
Analytical Techniques and Detection Limits
Updates to advisory concentrations, thresholds, or criteria for environmental matrices by regulatory agencies is creating a demand for state-of-the-art instrumentation that can achieve parts per quadrillion (ppq) concentrations. Achieving these detection limits is quite challenging considering the gold standard for target PFAS analysis is liquid chromatography tandem mass spectrometry (LC/MS/MS), which typically has detection limits in the parts per trillion (pptr) range. The target PFAS approach uses LC/MS/MS to quantify a finite known list of analytes. Additional analytical techniques are available for the analysis of PFAS precursors, nontarget PFAS compounds, total fluorine, and total organic fluorine. These techniques can provide additional information that may help with site characterization and source fingerprinting. Understanding the full extent of PFAS contamination (targets, nontargets, precursors, total fluorine, and organic fluorine) has important implications for PFAS remediation efforts. Merely focusing on target PFAS without considering the full suite of contamination could inadvertently result in further biotransformation and concentration increases through time resulting in further liability and restoration efforts.
PFAS compounds can be directly released into the environment, or they can be biotransformed in the environment by converting a precursor compound or coexisting contaminant or they may be coreleased with target PFAS (Wang and others, 2005). Common precursor compounds include FTOH and fluorotelomer sulfonates (FTS; refer to sec. 3 for more precursor examples); for example, the precursor 8:2 FTOH can be biotransformed into the PFAS terminal end products PFOA and perfluorohexanoic acid (PFHxA). Many of the precursors are not measured using the targeted approach nor does this approach measure the potential for PFAS formation from precursor transformations. The total oxidizable precursor assay (TOP) can be useful for understanding PFAS transformation of precursors in an environmental sample. The TOP assay simulates accelerated weathering of PFAS compounds through an oxidation reaction. TOP requires the sample to be collected in duplicate and analyzed twice. The first analysis uses the targeted techniques using LC/MS/MS to determine the “baseline” concentrations of the target analytes. Then the TOP assay alters the PFAS precursors through oxidation to form the terminal end products that can then be measured using the targeted approach with LC/MS/MS. The difference between the pre- and postoxidation concentrations of PFAS estimates the maximum presence and concentration of precursors as well as the potential for environmental or biological transformation of PFAS precursor compounds. The TOP assay methods are commercially available and can be applied to a variety of complex environmental matrices.
There are thousands of PFAS, but current target methods only quantify roughly 40 analytes (table 6). Nontarget analysis or suspect screening can scan for additional unknown PFAS using LC/MS with quadrupole time-of-flight mass spectrometry (Q-TOF, QTOF/MS, or LC/MS-qTOF). This high-resolution technology provides a more comprehensive characterization of PFAS contamination by screening for known PFAS and new or unknown analytes. To identify unknown PFAS, accurate masses of molecular ions, fragment ions, isotope patterns, reference standards (if available), additional chromatographic properties, and the use of mass spectral libraries are used. This technology is commercially available and can lead to a better understanding of PFAS contamination, the discovery of novel PFAS, measuring remediation success, and source fingerprinting.
PFAS Fingerprinting Methods
Source apportionment and fingerprinting methods such as computer-generated models, concentration ratios, or branched and linear isomers have been used to help identify contamination sources and contributions in environmental media and biological matrices. The EPA developed a few successful computer models for the source apportionment of PFAS which include UNMIX, positive matrix factorization, and principal component analysis-multiple linear regression (Lin and others, 2021; Zhong and others, 2021; Wallis and others, 2023). The computer models are not often used with biological media unless biological measurements have been made to help reconstruct exposure sources (Wallis and others, 2023). In addition, compound ratios are frequently used to help elucidate sources in biological matrices and environmental media. Ratios of PFOS relative to long-chain PFCAs (PFOS/LC–PFCA) or the ratios between total PFSAs to total PFCAs (PFSA/PFCA) can be used to help identify local or distant sources; for example, the ratio of PFOS/LC–PFCA has been used as a distance tracer between direct (local) emission sources (PFOS/LC–PFCA>1) in close proximity to industrialization compared to remote areas impacted only by atmospheric deposition (PFOS/LC–PFCA<1; Ali and others, 2021; Valsecchi and others, 2021). Other ratios may account for precursor degradation suggesting that certain PFCA ratio pairs above one could indicate long-range atmospheric transport; for instance, the atmospheric degradation of FTOH results in even- and odd-chained PFCAs (Langberg and others, 2022). Examples include the degradation of 8:2 FTOH into PFOA (C8) and PFNA (C9) (PFNA/PFOA), 10:2 FTOH [PFUnDA (C11)/PFDA (C10)] and 12:2 FTOH [PFTrDA (C13)/PFDoDA (C12)].
PFAS source apportionment and tracking methods should be considered preliminary since a comprehensive understanding of sources and releases is still under development; however, in some cases these methods can provide clear forensic information when considered within a site-specific conceptual model (Charbonnet and others, 2021).
Several studies have investigated general compositional characteristics to differentiate between unique AFFF, food packaging materials, WWTPs, and electrochemical fluorination processes (Moody and Field, 1999; Benotti and others, 2020; Ruyle and others, 2021a; Ruyle and others, 2021b; Helmer and others, 2022); for instance, the predominance of PFOS, PFHxS, PFNA and 6:2 Cl-PFESA may suggest a sizable contribution of historical AFFF formulations whereas the fluorotelomer-based AFFF are dominated by the fluorotelomers (for example, 6:2, 4:2, and 8:2 FT; Moody and others, 2003; Anderson and others, 2016). Other studies suggest short-chain PFCAs have been associated with hydraulic fluid formulations whereas PFTrDA, PFTeDA, PFHxDA, and PFECHs have been associated with microwave popcorn bags and fast-food packaging (Zafeiraki and others, 2014; Liu and others, 2019).
The total organic fluorine (TOF) concentrations in a sample are typically measured using combustion ion chromatography, although other technologies are being developed (e.g., proton-induced gamma-ray emission). TOF is a broadly used term for absorbable organic fluorine (AOF) in aqueous samples and extractable organic fluorine in solid matrices. This technology allows for the determination of the presence of other total inorganic and organic forms of fluorine in an environmental sample. TOF is a good screening methodology to capture all PFAS in a single measurement that is followed up with nontarget analysis methods to identify the unknowns; however, the disadvantages include the challenges in translating TOF into risk assessments and the assumption that all organofluorine substances are PFAS. The EPA is currently working on draft EPA Method 1621 for AOF (EPA, 2022b), in the meantime, AOF and extractable organic fluorine methods are commercially available through contract laboratories.
10. PFAS Concentrations in Environmental Media
PFAS concentrations in environmental media are presented in the following paragraphs to provide context for matrix-specific results although this is not a comprehensive review. The reader is encouraged to perform an updated search of PFAS concentrations in matrices of interest to their site or study including any regional values or trends. When interpreting concentrations and compositional profiles, it is important to understand the important factors that include data quality, target PFAS lists, precursors and degradation products, temporal effects, spatial effects, proper sampling techniques, baseline considerations (sec. 2), and weight of evidence (ITRC, 2023). The PFAS fingerprinting methods (sec. 7) may provide additional context for determining sources in a particular area.
PFAS Concentrations in the Atmosphere
Several of the major classes of PFAS (for example PFAAs, FASAs, FTOHs) have been measured in ambient air with concentrations ranging from 1 to 20 picograms per cubic meter (pg/m3) and reaching concentrations of 900,000 pg/m3 near large industrial facilities (Radi and others, 2022). Total PFCAs and PFSAs in global air ranged from 0.128 to 781 and 6.85 to 124 pg/m3, respectively (Saini and others, 2023). The average concentrations of total PFCAs at urban, rural, polar, and background sites were 152, 208, 34.5, and 65.0 pg/m3, respectively. Maximum PFCAs were measured over a rural site in the country of Colombia. PFHxA contributed ≥35 percent to the total PFCAs at more than 50 percent of the global sites. PFOS followed by PFHxS dominated the total PFSAs in global air, with the maximum concentrations measured over Little Fox Lake, Yukon, Canada (62.3 pg PFOS/m3, 28.0 pg PFHxS/m3; Saini and others, 2023).
PFAS Concentrations in Soil and Sediment
A 2020 metadata analysis compared soil samples collected worldwide from background sites (no known sources, no direct source impacts), primary-source sites (PFAS manufacturing, usage, or disposal sites), and secondary-source sites (indirect contamination from PFAS-contaminated biosolids or irrigation water); the study also presents PFAS depth (<1 meter [m] to 37 m subsurface) distributions (Brusseau and others, 2020). Background soil sampled from more than 1,400 sampling locations worldwide had reported total PFAS concentrations ranging from below the detection limit to 237 microgram per kilogram (µg/kg; parts per billion [ppb]) with maximum PFOS and PFOA concentrations ranging from 0.003 to 162 µg/kg and 0.01 to 124 µg/kg, respectively (Brusseau and others, 2020). Soil samples collected from more than 1,000 primary-source sites worldwide had maximum reported PFOS and PFOA concentrations ranging from 0.4 to 460,000 µg/kg and 2 to 50,000 µg/kg, respectively. Secondary-source sites contained maximum reported PFOS and PFOA concentrations ranging from 0.4 to 5,500 µg/kg and 0.8 to 2,531 µg/kg, respectively. Soil collected from contaminated sites are generally orders-of-magnitude higher than background concentrations. Shallow soil depths consisted mainly of long-chain PFAS, whereas deeper depths consisted mainly of the short-chain compounds. Observed PFAS concentrations decreased exponentially with depth, but this also indicates considerable retention of PFAS in the vadose zone through decadal timeframes (Brusseau and others, 2020).
PFAS Concentrations in Surface Water
Podder and others (2021) completed a metadata analysis of global concentrations of PFAS in surface water samples collected after 2010 that ranged from nondetect (ND) to 578,970 ng/L. The maximum concentration was for PFOAs collected near an industrial point source in China. PFOS (ND–1,650 ng/L) and PFOA (ND–578,970 ng/L) were detected in surface waters globally; however, their concentrations were tenfold higher in China, Asia, and Europe compared to North and South America. Between the years 1998 and 2019, PFOA and PFOS concentrations in surface water collected across the United States ranged from 8.7 to 113 ng/L and 19 to 170 ng/L, respectively. In response to regulations, a general decrease in PFOS was observed with time; however, a substantial increase in the usage of short-chain PFAS, as well as a global shift in PFOS usage from the western to eastern part of the world was noted (Podder and others, 2021). Similarly, a meta-analysis examining global trends from 2004 to 2020 in wastewater PFAS concentrations revealed short-chain PFAS increased whereas long-chain PFAS concentrations, including PFOA, PFOS and PFDA, have not changed significantly with time (Cookson and Detwiler, 2022). PFOS and PFOA concentrations ranged from below the limit of detection to 5,663 and 2,746 ng/L, respectively. These results suggest precursor loads can influence effluent concentrations and conventional WWTPs do not effectively remove PFAS.
PFAS Concentrations in Fish
A recent market basket study evaluated domestic and imported finfish and shellfish for PFAS concentrations (Ruffle and others, 2020). Finfish consisted of marine and freshwater species and included skinless fillets, skin-on fillets, gutted whole fish, and composites. Total PFAS in finfish collected in the United States ranged from ND to 21.5 ng/g wet weight (w.w.) whereas imported fish had concentrations <1 ng/g (w.w.); however, this study did not compare marine versus freshwater species, domestic versus imported, or skin-on versus skinless fillets. Another survey examined skin-on fillets from freshwater fish collected from 164 urban rivers across the United States and 157 nearshore sites within the Great Lakes (Stahl and others, 2014). PFOS was the predominant compound in all fish analyzed and the range of PFAS concentrations were similar between urban rivers (n=682; <method detection limit [MDL]–127 ng/g w.w.) and the Great Lakes (n=423; <MDL–80 ng/g w.w.). Multiple datasets of PFAS in freshwater fish that were either wild caught or commercially sold at supermarkets across the United States from 2013 to 2015 were recently evaluated because of their source of dietary exposure in humans (Barbo and others, 2023). Median concentrations of PFAS in fish fillets from the Great Lakes and nationwide rivers and streams were 11.8 and 9.5 ng/g w.w., respectively. Additionally, Barbo and others (2023) determined the PFAS concentration in wild caught freshwater fish were nearly 300 times higher than commercially relevant species tested by the FDA in 2019–22. Trends in PFAS concentrations measured in predator fish for example Salvelinus namaycush [lake trout] and Sander vitreus [walleye]) collected from the Great Lakes are generally declining with time with the exception of Lakes Superior and Erie, which are exhibiting stable or increasing trends (Point and others, 2021). Fish consumption advisories triggered by PFOS concentrations in the edible muscle tissue (2–200 ppb) have been issued for several states in the United States. Additionally, states with available consumption advisories have also issued ‘do not eat’ advisories for the general public for fish tissues containing PFOS concentrations ranging from 159 to 800 ppb. The wide range in the PFOS concentrations that trigger either consumption or “do not eat” advisories are because of the lack of regulations, toxicity data, and the use of different endpoints in the risk assessment process.
PFAS Concentrations in Upper Trophic Levels
PFOS and long-chain PFCAs were also the most frequently reported PFAS in marine and terrestrial apex predators (Chen and others, 2021b). Concentrations of PFOS in the liver of terrestrial apex predators (for example Ursus maritimus [polar bear]) that feed mainly on marine life (<MDL–3,270 nanogram per gram [ng/g] w.w.) were significantly higher than those in pinnipeds (<MDL–1,503 ng/g w.w.), cetaceans (0.30–1,213 ng/g w.w.) and other terrestrial apex predators (for example wolf) that do not feed mainly on marine life (1.41–985 ng/g w.w.). Extremely high PFOS concentrations have been reported in the livers of Otus lettia (collared scops owl; 11,300 ng/g w.w.) from South Korea and Cyanistes caeruleus (blue tits; 11,359 ng/g w.w.) near a PFOS production site in Europe. Among cetaceans, the highest liver concentrations of PFOS were reported for Sousa chinensis (Indo-Pacific humpback dolphins; 1,180 ng/g w.w.) in the Pearl River Delta, China, and Phocoena phocoena (harbor porpoise; 1,213 ng/g w.w.) from the Baltic Sea. It was also noted that PFOS concentrations in apex predators near industrial areas were significantly higher than remote regions, and predators from the Arctic had higher concentrations than those in the Antarctic; furthermore, eastern polar bears and their prey found closer to Russia and the White Sea and Barents Sea generally had higher concentrations than their western (Greenland) counterparts (Blévin and others, 2020; Chen and others, 2021b). These concentrations provide a global context to measured PFAS concentrations in biota. In addition, Table 7 provides examples of observed concentrations for various natural resources in the United States.
Table 7.
Examples of per- and polyfluoroalkyl substances (PFAS) concentrations measured in various natural resources across the United States.[Concentrations are reported in nanograms per gram wet weight for tissues or nanograms per liter for plasma and blood. Refer to table 1 for definitions of the constituents (PFSA, PFCA, PFNA, PFOS, PFOAA). --, no available data; MDL, method detection limit; ng/g, nanogram per gram; w.w., wet weight; LOD, limit of detection: <, less than; ∑xxPFAS, sum of x number of individual PFAS.]
Available Guidance Values
At the time of publication, the following information was available as guidance values, standards, or screening values (tables 8–12). Human, animal, and plant health are interdependent and bound to the health of the ecosystems; thus, changes in one environmental media may signal changes of other ecosystem goods and services (Keeler and others, 2012; Bateman and others, 2023; Vossler and others, 2023). Incorporating screening levels and values may improve decision-making and increase the weight of evidence. This section provides available guidance values and screening levels that are protective of the environmental media. These values can be used with risk assessments to characterize the nature and magnitude of health risks to humans and ecological receptors. We encourage the reader to check for updates to the following information for the state or region where their assessment area is located.
Table 8.
Examples of available screening levels and environmental quality standards (EQS) for perflurorooctane sulfonate (PFOS) and perfluorooctanoic acid (PFOA) in environmental media.[Refer to table 1 for definitions of the constituents (PFOS, PFOA, GenX, PFBS). pptr, part per trillion; ppb, part per billion; kg, kilogram; ppm, part per million; HA, health advisory; LOAEL, lowest-observed-adverse-effect level]
Media | Screening level or environmental quality standards (EQS) | Calculation approach | Source |
---|---|---|---|
Groundwater or potential source of drinking water | 40 pptr for PFOS or PFOA | Screening levels are typically based on default exposure parameters and factors that represent reasonable maximum exposure (RME) conditions for long-term/chronic exposures and normally are based on the methods recommended in EPA’s Risk Assessment Guidance for Superfund, Part B Manual (U.S. Environmental Protection Agency [EPA], 1991) and Soil Screening Guidance documents (EPA, 1996, 2002) | EPA, 2023 |
Drinking water lifetime health advisory | PFOA: 0.004 pptr (interim) | The lifetime health advisory for GenX chemicals used a chronic RfD from the final EPA toxicity assessment (EPA, 2021) based on the critical effect of adverse liver effects in adults (parental females) from a subchronic study (53–64 day exposure). In the assessment, a 10X UFS (subchronic-to-chronic exposure duration extrapolation uncertainty factor) for subchronic to chronic exposure was applied to derive the chronic reference dose, RfD (EPA, 2021). Because the critical effect identified for GenX chemicals is in adults, the HA applies to chronic exposure scenarios. The HA was based on exposure to lactating women, an adult life stage with the greatest drinking water intake rate. Application of the GenX chemicals HA to a shorter-term risk assessment scenario would provide a conservative, health protective approach in the absence of other information. | EPA, 2022 |
PFOS: 0.02 pptr (interim) | |||
GenX: 10 pptr (final) | |||
PFBS: 2,000 pptr (final) | |||
Surface water | PFOS: 6.8 ppb | Several cumulative distribution functions were fit to the data using regression methods and the best model was selected based on goodness-of-fit. The log normal model provided the best fit for the data and the 5th percentile of the species-sensitivity distribution (SSD) plot is 6.8 μg/L, with lower and upper confidence limits of 4.2 and 11 μg/L, respectively. | Environment and Climate Change Canada, 2018 |
Surface (marine) waters | PFOS: 7.2 ppb | Unless otherwise specified, the EQS parameter expressed as an annual average value (AA-EQS) applies to the total concentration of all isomers. Inland surface waters encompass rivers and lakes and related artificial or heavily modified water bodies. | European Chemicals Agency, 2023 |
Inland (freshwater) waters | PFOS: 36 ppb | Unless otherwise specified, the EQS parameter expressed as an annual average value (AA-EQS) applies to the total concentration of all isomers. Inland surface waters encompass rivers and lakes and related artificial or heavily modified water bodies. | European Chemicals Agency, 2023 |
Soil | PFOA: 0.915 ppb | Soil screening levels (SSLs) are either back-calculated from protective risk-based groundwater concentrations or based on maximum concentration levels (MCLs). The SSLs were designed for use during the early stages of a site evaluation when information about subsurface conditions may be scant. Because of this constraint, the equations used are based on conservative, simplifying assumptions about the release and transport of contaminants in the subsurface. Migration of contaminants from soil to groundwater can be envisioned as a two-stage process: (1) release of contaminant in soil leachate and (2) transport of the contaminant through the underlying soil and aquifer to a receptor well. The SSL methodology considers both of these fate and transport mechanisms. | Interstate Technology and Regulatory Council, 2022 |
PFOS: 0.038 ppb | |||
Soil (primary consumer: herbivorous mammals) | 2.2 mg PFOS / kg dry soil | The method used to calculate the soil quality guidelines to protect these consumers is found in Canadian Council of Ministers of the Environment (CCME, 2006). For herbivorous mammals the lowest effects dose for the primary consumer (ED1c) of 0.1086 mg/kg bw/day (from Thomford, 2002), was divided by an uncertainty factor of 2 according to methods described in the CCME protocol (2006) and based on the available data to obtain a daily threshold effects dose (DTED) of 0.0543 mg/kg bw/day. | Environment and Climate Change Canada, 2017 |
Soil (primary consumer: herbivorous bird) | 5.1 mg PFOS / kg dry soil | Environment and Climate Change Canada, 2017 | |
Soil (secondary consumer: insectivorous mammal) | 0.012 mg PFOS / kg dry soil | The method used to calculate the soil quality guidelines to protect these consumers is found in CCME (2006). The model developed to represent this food chain and to derive the Federal Soil Quality Guidelines for secondary consumer (FSQG2C) is similar to the one used in deriving FSQG1C, where 1C is for a primary consumer; however, to account for biomagnification of PFOS from contaminated soil and food to the predator, the bioaccumulation factor from soil to prey (BAF2) was used in addition to the bioconcentration factor soil to primary consumer (BCF1). | Environment and Climate Change Canada, 2017 |
Soil (secondary consumer: omnivorous mammal) | 0.17 mg PFOS / kg dry soil | ||
Soil (secondary consumer: omnivorous bird) | 0.33 mg PFOS / kg dry soil | ||
Soil (tertiary consumer: carnivorous mammal) | 2.6 mg PFOS / kg dry soil | ||
Soil (tertiary consumer: Omnivorous mammal) | 0.63 mg PFOS / kg dry soil | ||
Piscivorous mammals | PFOS: 4.6 ppb | Tolerable daily intake (TDI) values were calculated as the critical toxicity values from nine studies divided by an uncertainty factor of 100. The minimum TDI was then divided by the maximum food intake to body weight ratio for American mink (Neovison vison). | Environment and Climate Change Canada, 2017 |
Piscivorous birds | PFOS: 8.2 ppb | LOAEL dose rate in northern bobwhite with applied uncertainty factor of 100 to produce a TDI. The TDI was then divided by the maximum avian food intake to body weight ratio for Wilson’s storm-petrel (Oceanites oceanicus). | Environment and Climate Change Canada, 2017 |
Bird egg | PFOS: 1.9 ppm | LOAEL for quail egg yolk divided by a safety factor of 10, then converted to whole egg concentrations using yolk: albumin ratio and egg density. | Environment and Climate Change Canada, 2017 |
Fish tissue | PFOS: 9.4 ppm | Water quality guideline multiplied by the geometric mean of by bioaccumulation factors (BAFs) for bluegill sunfish (Lepomis macrochirus) and carp (Cyprinidae) | Environment and Climate Change Canada, 2018 |
Freshwater fish whole-body | PFOA: 6.10 ppm (Draft) | Water column concentration multiplied by BAFs | EPA, 2022c |
PFOS: 6.75 ppm (Draft) | |||
Freshwater fish muscle | PFOA: 0.125 ppm (Draft) | Water column concentration multiplied by BAFs | EPA, 2022c |
PFOS: 2.91 ppm (Draft) | |||
Freshwater invertebrate whole-body | PFOA: 1.11 ppm (Draft) | Water column concentration multiplied by BAFs | EPA, 2022c |
PFOS: 0.937 ppm (Draft) | |||
Biota | PFOS: 9.1 ppb | Unless otherwise indicated, the biota EQS relate to fish. An alternative biota taxon, or another matrix, may be monitored instead, as long as the EQS applied provides an equivalent level of protection. | European Chemicals Agency, 2023 |
Table 9.
Empirically derived toxicity reference values.[Modified from the Interstate Technology and Regulatory Council (2021a). Refer to table 1 for definitions of PFAS. NOAEL, no-observed-adverse-effect level; TRV, toxicity reference value; LOAEL, lowest-observed-adverse-effect-level; mg/kg/d, milligram per kilogram per day; GD, gestation days; --, no available data]
Organism common name (scientific name) | Duration | Effect/endpoint | NOAEL TRV (mg/kg/d) | LOAEL TRV (mg/kg/d) | Source |
---|---|---|---|---|---|
Rat (Rattus norvegicus) | 14 days | Hepatic | 0.1 | 1 | ATSDR, 2021 |
Rat (Rattus norvegicus) | 2 years | Liver function; reproduction | 0.1 | 0.4 | Thomford, 2002 |
Rat (Rattus norvegicus) | GD 6–15 | Maternal weight loss and developmental toxicity; reduced birth weight | 1 | 5 | Gortner, 1980, as reported by ITRC, 2021a |
Rat (Rattus norvegicus) | GD 2–20 | Neonatal mortality | -- | 25 | Grasty and others, 2003 |
Rat (Rattus norvegicus) | 6 weeks before mating through day 4 of lactation | Gestation length and pup viability | 0.4 | 0.8 | Luebker and others, 2005 |
Rat (Rattus norvegicus) | 2 generation | Gestation length and pup viability; reduced birth weight | 0.1 | 0.4 | Christian and others, 1999 as reported by ITRC, 2021a |
Rabbit (Oryctolagus cuniculus) | GD 6–20 | Maternal weight gain | 0.1 | 1 | Case and others, 2001 |
Rabbit (Oryctolagus cuniculus) | GD 6–20 | Birth weight and delayed ossification | 1 | 2.5 | Case and others, 2001 |
Rat (Rattus norvegicus) | 2 years | Reproduction | 1.5 | 15 | ATSDR, 2021 |
Rat (Rattus norvegicus) | 90 days | Reduced weight gain | 1.9 | 6.4 | Perkins and others, 2004 |
Rat (Rattus norvegicus) | 104 weeks | Reduced weight gain | 1.6 | 14.2 | Sibinski, 1987, as reported by EFSA, 2008 |
Rat (Rattus norvegicus) | 2 generations | Decreased body weight | 1 | 3 | Butenhoff and others, 2004 |
Rat (Rattus norvegicus) | 2 generations | Reproductive function | 30 | -- | Butenhoff and others, 2004 |
Rat (Rattus norvegicus) | 2 generations | Sexual maturation | 10 | 30 | Butenhoff and others, 2004 |
Mouse (Mus musculus) | Day 1 pregnancy until birth | Postnatal survival | 3 | 5 | Lau and others, 2006 |
Mouse (Mus musculus) | -- | Postnatal weight gain of pups | -- | 3 | Wolf and others, 2007 |
Rhesus monkey (Macaca mulatta) | 90 days | Survival | 10 | 30 | Goldenthal, 1978, as reported by EFSA, 2008 |
Rat (Rattus norvegicus) | Females 14 days; males 42 days | Reproduction | 1 | -- | Butenhoff and others, 2009 |
Table 10.
Theoretically derived toxicity reference values published by Zodrow and others (2021).[Refer to table 1 for definitions of chemicals. Toxicity reference values (TRVs) were calculated using the EcoSSL Methodology. The no-observed-adverse-effect levels (NOAEL) and lowest-observed-adverse-effect-level (LOAEL) selected for inclusion in the TRV datasets were based on studies with exposure durations of 3 days or greater and survival, growth, or reproduction endpoints. mg/kg/d, milligram per kilogram per day]
Table 11.
Aquatic risk-based screening levels derived by Zodrow and others (2021) for muskrat (Ondatra zibethicus), little brown bat (Myotis lucifugus), harbor seal (Phoca vitulina), mink (Mustelidae), red-winged blackbird (Agelaius phoeniceus), tree swallow (Tachycineta bicolor), and brown pelican (Pelecanus occidentalis).[Refer to table 1 for definitions of constituents. Toxicity reference values (TRV) calculated based on the EcoSSL Methodology (Divine and others, 2020). The no-observed-adverse-effect levels (NOAEL) and lowest-observed-adverse-effect-level (LOAEL) selected for inclusion in the TRV datasets were based on studies with exposure durations of 3 days or greater and survival, growth, or reproduction endpoints. RBSL, risk-based screening levels; mg/kg, milligram per kilogram; E, 10 to the power of; --, no data; mg/L milligram per liter]
Table 12.
Terrestrial risk-based screening levels derived by Zodrow and others (2021) for meadow vole (Microtus pennsylvanicus), short-tailed shrew (Blarina brevicauda), little brown bat (Myotis lucifugus), long-tailed weasel (Mustela frenata), American goldfinch (Spinus tristis), house wren (Troglodytes aedon), and red-tailed hawk (Buteo jamaicensis).[Toxicity reference values (TRV) calculated based on the EcoSSL Methodology (Divine and others, 2020). The no-observed-adverse-effect level (NOAEL) and the lowest-observed-adverse-effect-level (LOAEL) selected for inclusion in the TRV datasets were based on studies with exposure durations of 3 days or greater and survival, growth, or reproduction endpoints. RBSL, risk-based screening levels; mg/kg, milligram per kilogram; E, 10 to the power of; --, no data; mg/L milligram per liter]
Drinking Water
Water standards and guidance values for ~24 PFAS (where available) have been issued by individual states, the EPA, and internationally (ATSDR, 2021; ITRC, 2022f, d). As of June 2022, the EPA issued interim lifetime health advisories for drinking water that ranged from 0.004 pptr for PFOA to 2,000 pptr for PFBS (EPA, 2022d). These 2022 interim values will remain available as the EPA finalizes the March 2023 proposed National Primary Drinking Water Regulation for six PFAS in public water systems. The proposed nonenforceable MCLG are 0 pptr for PFOA and PFOS. The proposed enforceable MCL are 4.0 pptr for PFOA and PFOS. For PFNA, PFHxS, PFBS, and HFPO-DA, the EPA is proposing to use a hazard index of 1 for both the nonenforceable MCLGs and enforceable MCLs. Several states have issued their recommended screening values, MCLs, and action levels for drinking water that are several orders of magnitude higher (low pptr to ppm range) than the 2022 EPA Interim Lifetime Health Advisories (ITRC, 2022f).
Surface Water (Including Sediment)
Screening levels have been outlined by the European Chemicals Agency (2023) and Environment and Climate Change Canada (2017, 2018) for marine and inland surface waters. Table 8 provides examples of available screening levels and environmental quality standards (EQS). These organizations provide EQS for PFOS that range from 6.8 to 7.2 ppb for marine surface waters and 36 ppb for freshwater.
The EPA has provided EQS values for PFOS and PFOA in freshwater biota in a 2022 draft. These values, ranging from 0.937 to 6.75 ppm for PFOS and 0.125 to 6.10 ppm for PFOA, can be found in table 8 along with a brief outline of the calculation approach used by the EPA to determine these data. Soil screening levels have also been reported by ITRC for PFOA and PFOS at 0.915 ppb and 0.038 ppb, respectively. Table 8 also provides an overview of the calculation approaches used by the agencies to determine the EQS for each matrix.
Soil Standards/Screening Values
In addition to ITRC’s soil screening levels, the Environment and Climate Change Canada has published soil guidance values for PFOS in biota. These values, found in table 8, are reported in milligrams per kilogram (mg/kg) and demonstrate a wide range of effects in different ecological trophic levels. These EQS values range as high as 5.1 mg/kg in a primary consumer to as low as 0.012 mg/kg and 0.63 mg/kg in secondary and tertiary consumers, respectively.
Toxicity Reference Values and Risk-based Screening Levels
Available empirically and theoretically derived toxicity reference values have been summarized in tables 9–10. Additional values may be obtained from the literature (ATSDR, 2021; ITRC, 2021a). Tables 11–12 summarize aquatic and terrestrial risk-based screening levels (RBSL) developed for 23 PFAS using surrogate receptors (Zodrow and others, 2021). For wildlife receptors, the no-observed-adverse-effect level RBSLs ranged from 0.013 to 340 ppm for soil, 0.0014 to 370 ppm for sediment, and 0.000075 to 1,600 ppm for surface water. The chronic RBSLs ranged from 0.00022 to 3.4 ppm for aquatic life and the no‐observed‐effect concentration screening levels ranged from 0.084 to 642 ppm and 1 to 50 ppm, for terrestrial plants and soil invertebrates, respectively.
11. Summary and Key Considerations
The physicochemical properties of per- and polyfluoroalkyl substances (PFAS) have resulted in their widespread usage in industrial and consumer applications, environmental stability, and extensive pervasiveness. These same characteristics also make it challenging to select PFAS-free field gear and collection apparatus, determine background or baseline concentrations, and determine specific sources. PFAS research is rapidly evolving to advance the knowledge on recommended sampling procedures, analytical methods, source apportionment, and toxicity effect levels. As such, it is best practice to consult the analytical laboratory and develop a Quality Assurance Project Plan before field sampling to eliminate potential sample contamination. Selecting a suite of analytical tools may help to identify sources and estimate the full extent of PFAS contamination. The science and knowledge of PFAS and effects to natural resources is constantly advancing. At the time of this publication, screening values, criteria, and toxicity reference values were available from several states, the EPA, and the peer-reviewed literature; however, it is important to note that this guidance document is not comprehensive and will likely change because of the rapid advancement of PFAS science. Users are highly encouraged to consult the literature and references provided herein for updated information before using the screening values, background concentrations, and models provided for interpretation of site-specific data.
References Cited
3M, 2021, PFAS history: 3M web page, accessed December 12, 2022, at https://www.3m.com/3M/en_US/pfas-stewardship-us/pfas-history/.
3M Global EHS Laboratory, 2021, Summary of physical/chemical and environmental parameters for PFAS—Subject to interim Special Order by Consent No. 20-086CWP/AP/GW/HW/DW/SW, paragraph 37(J)(3): 3M, Laboratory Request and Project Number E21-0037: 3M Global EHS Laboratory report E21–0037, 5 p., 4 attachments, accessed March 8, 2023, at https://semspub.epa.gov/work/HQ/100002785.pdf.
Adu, O., Ma, X., and Sharma, V.K., 2023, Bioavailability, phytotoxicity and plant uptake of per-and polyfluoroalkyl substances (PFAS)—A review: Journal of Hazardous Materials, v. 447, p. 130805. [Also available at https://doi.org/10.1016/j.jhazmat.2023.130805.]
Ahrens, L., and Bundschuh, M., 2014, Fate and effects of poly- and perfluoroalkyl substances in the aquatic environment—A review: Environmental Toxicology and Chemistry, v. 33, no. 9, p. 1921–1929. [Also available at https://doi.org/10.1002/etc.2663.]
Ahrens, L., Hedlund, J., Dürig, W., Tröger, R., and Wiberg, K., 2016, Screening of PFASs in groundwater and surface water: Swedish Environmental Protection Agency, prepared by the Swedish University of Agricultural Sciences, 41 p. [Also available at https://pub.epsilon.slu.se/13236/1/ahrens_l_etal_160330.pdf.]
Ahrens, L., Shoeib, M., Harner, T., Lee, S.C., Guo, R., and Reiner, E.J., 2011a, Wastewater treatment plant and landfills as sources of polyfluoroalkyl compounds to the atmosphere: Environmental Science & Technology, v. 45, no. 19, p. 8098–8105. [Also available at https://doi.org/10.1021/es1036173.]
Ahrens, L., Siebert, U., and Ebinghaus, R., 2009, Total body burden and tissue distribution of polyfluorinated compounds in harbor seals (Phoca vitulina) from the German Bight: Marine Pollution Bulletin, v. 58, no. 4, p. 520–525. [Also available at https://doi.org/10.1016/j.marpolbul.2008.11.030.]
Ahrens, L., Yeung, L.W.Y., Taniyasu, S., Lam, P.K.S., and Yamashita, N., 2011b, Partitioning of perfluorooctanoate (PFOA), perfluorooctane sulfonate (PFOS) and perfluorooctane sulfonamide (PFOSA) between water and sediment: Chemosphere, v. 85, no. 5, p. 731–737. [Also available at https://doi.org/10.1016/j.chemosphere.2011.06.046.]
Ali, A.M., Sanden, M., Higgins, C.P., Hale, S.E., Alarif, W.M., Al-Lihaibi, S.S., Ræder, E.M., Langberg, H.A., and Kallenborn, R., 2021, Legacy and emerging per- and polyfluorinated alkyl substances (PFASs) in sediment and edible fish from the Eastern Red Sea: Environmental Pollution, v. 280, p. 116935. [Also available at https://doi.org/10.1016/j.envpol.2021.116935.]
Allendorf, F., Berger, U., Goss, K., and Ulrich, N., 2019, Partition coefficients of four perfluoroalkyl acid alternatives between bovine serum albumin (BSA) and water in comparison to ten classical perfluoroalkyl acids: Royal Society of Chemistry, v. 21, p. 1852–1863. [Also available at https://doi.org/10.1039/C9EM00290A.]
Alvarez, D.A., Huckins, J.N., Petty, J.D., Jones-Lepp, T.L., Stuer-Lauridsen, F., Getting, D.T., Goddard, J.P., and Gravell, A., 2007, Tool for monitoring hydrophilic contaminants in water—Polar organic vhemical integrative sampler (POCIS), chap. 8 of Greenwood, R., Mills, G., and Vrana, B., eds., Passive sampling techniques in environmental monitoring, vol. 48 of Comprehensive analytical chemistry: Elsevier, p. 171–197.
Alvarez, D.A., 2010, Guidelines for the use of the semipermeable membrane device (SPMD) and the polar organic chemical integrative sampler (POCIS) in environmental monitoring studies: U.S. Geological Survey Techniques and Methods, book 1, chap. D4, 38 p. [Also available at https://doi.org/10.3133/tm1D4.]
Anderson, R.H., Long, G.C., Porter, R.C., and Anderson, J.K., 2016, Occurrence of select perfluoroalkyl substances at US Air Force aqueous film-forming foam release sites other than fire-training areas—Field-validation of critical fate and transport properties: Chemosphere, v. 150, p. 678–685. [Also available at https://doi.org/10.1016/j.chemosphere.2016.01.014.]
Agency for Toxic Substances and Disease Registry [ATSDR], 2021, Toxicological profile for perfluoroalkyls: Agency for Toxic Substances and Disease Registry web page, accessed September 7, 2023, at https://www.atsdr.cdc.gov/ToxProfiles/tp200.pdf.
Bangma, J.T., Bowden, J.A., Brunell, A.M., Christie, I., Finnell, B., Guillette, M.P., Jones, M., Lowers, R.H., Rainwater, T.R., Reiner, J.L., Wilkinson, P.M., and Guillette, L.J., Jr., 2017a, Perfluorinated alkyl acids in plasma of American alligators (Alligator mississippiensis) from Florida and South Carolina: Environmental Toxicology and Chemistry, v. 36, no. 4, p. 917–925. [Also available at https://doi.org/10.1002/etc.3600.]
Bangma, J.T., Ragland, J.M., Rainwater, T.R., Bowden, J.A., Gibbons, J.W., and Reiner, J.L., 2019, Perfluoroalkyl substances in diamondback terrapins (Malaclemys terrapin) in coastal South Carolina: Chemosphere, v. 215, p. 305–312. [Also available at https://doi.org/10.1016/j.chemosphere.2018.10.023.]
Bangma, J.T., Reiner, J.L., Botha, H., Cantu, T.M., Gouws, M.A., Guillette, M.P., Koelmel, J.P., Luus-Powell, W.J., Myburgh, J., Rynders, O., Sara, J.R., Smit, W.J., and Bowden, J.A., 2017b, Tissue distribution of perfluoroalkyl acids and health status in wild Mozambique tilapia (Oreochromis mossambicus) from Loskop Dam, Mpumalanga, South Africa: Journal of Environmental Sciences, v. 61, p. 59–67. [Also available at https://doi.org/10.1016/j.jes.2017.03.041.]
Barbo, N., Stoiber, T., Naidenko, O.V., and Andrews, D.Q., 2023, Locally caught freshwater fish across the United States are likely a significant source of exposure to PFOS and other perfluorinated compounds: Environmental Research, v. 220, p. 115165. [Also available at https://doi.org/10.1016/j.envres.2022.115165.]
Barmentlo, S.H., Stel, J.M., van Doom, M., Eschauzier, C., de Voogt, P., and Kraak, M.H.S., 2015, Acute and chronic toxicity of short chained perfluoroalkyl substances to Daphnia magna: Environmental Pollution, v. 198, p. 47–53. [Also available at https://doi.org/10.1016/j.envpol.2014.12.025.]
Bateman, I.J., Keeler, B., Olmstead, S.M., and Whitehead, J., 2023, Perspectives on valuing water quality improvements using stated preference methods: Proceedings of the National Academy of Sciences of the United States of America, v. 120, no. 18, p. e2217456120. [Also available at https://doi.org/10.1073/pnas.2217456120.]
Beach, S.A., Newsted, J.L., Coady, K., and Giesy, J.P., 2006, Ecotoxicological evaluation of perfluorooctanesulfonate (PFOS): Reviews of Environmental Contamination and Toxicology, v. 186, p. 133–174. [Also available at https://doi.org/10.1007/0-387-32883-1_5.]
Benotti, M.J., Fernandez, L.A., Peaslee, G.F., Douglas, G.S., Uhler, A.D., and Emsbo-Mattingly, S., 2020, A forensic approach for distinguishing PFAS materials: Environmental Forensics, v. 21, no. 3-4, p. 319–333. [Also available at https://doi.org/10.1080/15275922.2020.1771631.]
Blaine, A.C., Rich, C.D., Sedlacko, E.M., Hyland, K.C., Stushnoff, C., Dickenson, E.R.V., and Higgins, C.P., 2014, Perfluoroalkyl acid uptake in lettuce (Lactuca sativa) and strawberry (Fragaria ananassa) irrigated with reclaimed water: Environmental Science & Technology, v. 48, no. 24, p. 14361–14368. [Also available at https://doi.org/10.1021/es504150h.]
Blazer, V.S., Gordon, S.E., Walsh, H.L., and Smith, C.R., 2021, Perfluoroalkyl substances in plasma of smallmouth bass from the Chesapeake Bay Watershed: International Journal of Environmental Research and Public Health, v. 18, no. 11, p. 5881. [Also available at https://doi.org/10.3390/ijerph18115881.]
Blévin, P., Aars, J., Andersen, M., Blanchet, M.-A., Hanssen, L., Herzke, D., Jeffreys, R.M., Nordøy, E.S., Pinzone, M., de la Vega, C., and Routti, H., 2020, Pelagic vs coastal—Key drivers of pollutant levels in Barents Sea polar bears with contrasted space-use strategies: Environmental Science & Technology, v. 54, no. 2, p. 985–995. [Also available at https://doi.org/10.1021/acs.est.9b04626.]
Bots, J., De Bruyn, L., Snijkers, T., Van den Branden, B., and Van Gossum, H., 2010, Exposure to perfluorooctane sulfonic acid (PFOS) adversely affects the life-cycle of the damselfly Enallagma cyathigerum: Environmental Pollution, v. 158, no. 3, p. 901–905. [Also available at https://doi.org/10.1016/j.envpol.2009.09.016.]
Boudreau, T.M., Sibley, P.K., Mabury, S.A., Muir, D.G., and Solomon, K.R., 2003, Laboratory evaluation of the toxicity of perfluorooctane sulfonate (PFOS) on Selenastrum capricornutum, Chlorella vulgaris, Lemna gibba, Daphnia magna, and Daphnia pulicaria: Archives of Environmental Contamination and Toxicology, v. 44, no. 3, p. 307–313. [Also available at https://doi.org/10.1007/s00244-002-2102-6.]
Brusseau, M.L., Anderson, R.H., and Guo, B., 2020, PFAS concentrations in soils—Background levels versus contaminated sites: Science of the Total Environment, v. 740, p. 140017. [Also available at https://doi.org/10.1016/j.scitotenv.2020.140017.]
Brusseau, M.L., Guo, B., Huang, D., Yan, N., and Lyu, Y., 2021, Ideal versus nonideal transport of PFAS in unsaturated porous media: Water Research, v. 202, p. 117405. [Also available at https://doi.org/10.1016/j.watres.2021.117405.]
Buck, R.C., Franklin, J., Berger, U., Conder, J.M., Cousins, I.T., De Voogt, P., Jensen, A.A., Kannan, K., Mabury, S.A., and van Leeuwen, S.P.J., 2011, Perfluoroalkyl and polyfluoroalkyl substances in the environment—Terminology, classification, and origins: Integrated Environmental Assessment and Management, v. 7, no. 4, p. 513–541. [Also available at https://doi.org/10.1002/ieam.258.]
Bureau of Land Management, 2008, BLM natural resource damage assessment and restoration handbook: Bureau of Land Management report, rel. 1–1712, variously paged. [Also available at https://www.doi.gov/sites/doi.gov/files/migrated/restoration/library/upload/BLM_Handbook.pdf.]
Burkhard, L.P., 2021, Evaluation of published bioconcentration factor (BCF) and bioaccumulation factor (BAF) data for per- and polyfluoroalkyl substances across aquatic species: Environmental Toxicology and Chemistry, v. 40, no. 6, p. 1530–1543. [Also available at https://doi.org/10.1002/etc.5010.]
Bursian, S.J., Link, J.E., McCarty, M., and Simcik, M.F., 2021, The subacute toxicity of perfluorooctane sulfonate and/or perfluorooctanoic acid and legacy aqueous film-forming foams to Japanese quail (Coturnix japonica): Environmental Toxicology and Chemistry, v. 40, no. 3, p. 695–710. [Also available at https://doi.org/10.1002/etc.4684.]
Butenhoff, J.L., Chang, S.C., Ehresman, D.J., and York, R.G., 2009, Evaluation of potential reproductive and developmental toxicity of potassium perfluorohexanesulfonate in Sprague Dawley rats: Reproductive Toxicology (Elmsford, N.Y.), v. 27, no. 3-4, p. 331–341. [Also available at https://doi.org/10.1016/j.reprotox.2009.01.004.]
Butenhoff, J.L., Kennedy, G.L., Jr., Frame, S.R., O’Connor, J.C., and York, R.G., 2004, The reproductive toxicology of ammonium perfluorooctanoate (APFO) in the rat: Toxicology, v. 196, no. 1-2, p. 95–116. [Also available at https://doi.org/10.1016/j.tox.2003.11.005.]
Canadian Council of Ministers of the Environment [CCME], 2006, A protocol for the derivation of environmental and human health soil quality guidelines: Winnipeg, Manitoba, Canada, Canadian Council of Ministers of the Environment report, 186 p. [Also available at https://ccme.ca/en/res/a-protocol-for-the-derivation-of-environmental-and-human-health-soil-quality-guidelines-en.pdf.]
Casas, G., Martínez-Varela, A., Roscales, J.L., Vila-Costa, M., Dachs, J., and Jiménez, B., 2020, Enrichment of perfluoroalkyl substances in the sea-surface microlayer and sea-spray aerosols in the Southern Ocean: Environmental Pollution, v. 267, p. 115512. [Also available at https://doi.org/10.1016/j.envpol.2020.115512.]
Case, M.T., York, R.G., and Christian, M.S., 2001, Rat and rabbit oral developmental toxicology studies with two perfluorinated compounds: International Journal of Toxicology, v. 20, no. 2, p. 101–109. [Also available at https://doi.org/10.1177/109158180102000207.]
ChemicalBook, 2023, ChemicalBook search engine: ChemicalBook website, accessed March 8, 2023, at https://www.chemicalbook.com.
Charbonnet, J.A., Rodowa, A.E., Joseph, N.T., Guelfo, J.L., Jones, G.D., Higgins, C.P., Helbling, D.E., and Houtz, E.F., 2021, Environmental source tracking of per- and polyfluoroalkyl substances within a forensic context—Current and future techniques: Environmental Science & Technology, v. 55, no. 11, p. 7237–7245. [Also available at https://doi.org/10.1021/acs.est.0c08506.
Chen, H., Reinhard, M., Yin, T., Nguyen, T.V., Tran, N.H., and Yew-Hoong Gin, K., 2019, Multi-compartment distribution of perfluoroalkyl and polyfluoroalkyl substances (PFASs) in an urban catchment system: Water Research, v. 154, p. 227–237. [Also available at https://doi.org/10.1016/j.watres.2019.02.009.]
Chen, H., Zhang, C., Yu, Y., and Han, J., 2012, Sorption of perfluorooctane sulfonate (PFOS) on marine sediments: Marine Pollution Bulletin, v. 64, no. 5, p. 902–906. [Also available at https://doi.org/10.1016/j.marpolbul.2012.03.012.]
Chen, M., Zhu, L., Wang, Q., and Shan, G., 2021a, Tissue distribution and bioaccumulation of legacy and emerging per-and polyfluoroalkyl substances (PFASs) in edible fishes from Taihu Lake, China: Environmental Pollution, v. 268, p. 115887. [Also available at https://doi.org/10.1016/j.envpol.2020.115887.]
Chen, Y., Fu, J., Ye, T., Li, X., Gao, K., Xue, Q., Lv, J., Zhang, A., and Fu, J., 2021b, Occurrence, profiles, and ecotoxicity of poly- and perfluoroalkyl substances and their alternatives in global apex predators—A critical review: Journal of Environmental Sciences (China), v. 109, p. 219–236. [Also available at https://doi.org/10.1016/j.jes.2021.03.036.]
Christou, A., Papadavid, G., Dalias, P., Fotopoulos, V., Michael, C., Bayona, J.M., Pina, B., and Fatta-Kassinos, D., 2019, Ranking of crop plants according to their potential to uptake and accumulate contaminants of emerging concern: Environmental Research, v. 170, p. 422–432. [Also available at https://doi.org/10.1016/j.envres.2018.12.048.]
Conder, J.M., Hoke, R.A., Wolf, W., Russell, M.H., and Buck, R.C., 2008, Are PFCAs bioaccumulative? A critical review and comparison with regulatory criteria and persistent lipophilic compounds: Environmental Science & Technology, v. 42, no. 4, p. 995–1003. [Also available at https://doi.org/10.1021/es070895g.]
Cookson, E.S., and Detwiler, R.L., 2022, Global patterns and temporal trends of perfluoroalkyl substances in municipal wastewater—A meta-analysis: Water Research, v. 221, p. 118784. [Also available at https://doi.org/10.1016/j.watres.2022.118784.]
Cousins, I.T., DeWitt, J.C., Glüge, J., Goldenman, G., Herzke, D., Lohmann, R., Miller, M., Ng, C.A., Scheringer, M., Vierke, L., and Wang, Z., 2020, Strategies for grouping per- and polyfluoroalkyl substances (PFAS) to protect human and environmental health: Environmental Science. Processes & Impacts, v. 22, no. 7, p. 1444–1460. [Also available at https://doi.org/10.1039/D0EM00147C.]
D’Ambro, E.L., Pye, H.O.T., Bash, J.O., Bowyer, J., Allen, C., Efstathiou, C., Gilliam, R.C., Reynolds, L., Talgo, K., and Murphy, B.N., 2021, Characterizing the air emissions, transport, and deposition of per- and polyfluoroalkyl substances from a fluoropolymer manufacturing facility: Environmental Science & Technology, v. 55, no. 2, p. 862–870. [Also available at https://doi.org/10.1021/acs.est.0c06580.]
DENIX, 2019, DoD Environmental Laboratory Accreditation Program (DoD ELAP): Department of Defense web page, accessed June 1, 2023, at https://www.denix.osd.mil/edqw/accreditation/home/.
Denly, E., Occhialini, J., Bassignani, P., Eberle, M., and Rabah, N., 2019, Per- and polyfluoroalkyl substances in environmental sampling products—Fact or fiction?: Remediation Journal, v. 29, no. 4, p. 65–76. [Also available at https://doi.org/10.1002/rem.21614.]
Dennis, N.M., Karnjanapiboonwong, A., Subbiah, S., Rewerts, J.N., Field, J.A., McCarthy, C., Salice, C.J., and Anderson, T.A., 2020, Chronic reproductive toxicity of perfluorooctane sulfonic acid and a simple mixture of perfluorooctane sulfonic acid and perfluorohexane sulfonic acid to northern bobwhite quail (Colinus virginianus): Environmental Toxicology and Chemistry, v. 39, no. 5, p. 1101–1111. [Also available at https://doi.org/10.1002/etc.4703.]
Department of the Navy, 2023, DON’s strategic management response for PFAS: Department of the Navy web page, accessed June 1, 2023, at https://www.secnav.navy.mil/eie/Pages/PFAS_Home.aspx.
Dickman, R.A., and Aga, D.S., 2022, A review of recent studies on toxicity, sequestration, and degradation of per- and polyfluoroalkyl substances (PFAS): Journal of Hazardous Materials, v. 436, p. 129120. [Also available at https://doi.org/10.1016/j.jhazmat.2022.129120.]
Ding, G.H., Frömel, T., van den Brandhof, E.J., Baerselman, R., and Peijnenburg, W.J., 2012, Acute toxicity of poly- and perfluorinated compounds to two cladocerans, Daphnia magna and Chydorus sphaericus: Environmental Toxicology and Chemistry, v. 31, no. 3, p. 605–610. [Also available at https://doi.org/10.1002/etc.1713.]
Ding, G., and Willie, J.G.M., 2013, Physicochemical properties and aquatic toxicity of poly- and perfluorinated compounds: Critical Reviews in Environmental Science and Technology, v. 43, no. 6, p. 598–678. [Also available at https://doi.org/10.1080/10643389.2011.627016.]
Divine, C., Zodrow, J., Frenchmeyer, M., Dally, K., Osborn, E., and Anderson, P., 2020, Approach for assessing PFAS risk to threatened and endangered species: Department of Defense Strategic Environmental Research and Development Program final report, SERDP Project ER18–1653, variously paged. [Also available at https://serdp-estcp-storage.s3.us-gov-west-1.amazonaws.com/s3fs-public/project_documents/ER18-1653_Final_Report.pdf.]
Du, Z., Deng, S., Bei, Y., Huang, Q., Wang, B., Huang, J., and Yu, G., 2014, Adsorption behavior and mechanism of perfluorinated compounds on various adsorbents—A review: Journal of Hazardous Materials, v. 274, p. 443–454. [Also available at https://doi.org/10.1016/j.jhazmat.2014.04.038.]
Dykstra, C.R., Route, W.T., and Williams, K.A., 2021, Trends and patterns of perfluoroalkyl substances in blood plasma samples of bald eagle nestlings in Wisconsin and Minnesota, USA: Environmental Toxicology and Chemistry, v. 40, no. 3, p. 754–766. [Also available at https://doi.org/10.1002/etc.4864.]
Edelstein, K., 2021, Impacts of 2020 Colonial Pipeline rupture continue to grow: Fractracker Alliance web page, accessed October 14, 2022, at https://www.fractracker.org/2021/05/august-2020-colonial-pipeline-spill-in-north-carolina/#:~:text=Colonial%E2%80%99s%20response%20Colonial%20issued%2 0an%20update%20on%20the,Mecklenburg%20County%20officials%2C%20to%20continue%20the%20mitigation%20work.
Environment and Climate Change Canada, 2017, Canadian Environmental Protection Act, 1999—Federal environmental quality guidelines—Perfluorooctane sulfonate (PFOS): Government of Canada, Environment and Climate Change Canada, 25 p. [Also available at https://www.ec.gc.ca/ese-ees/38E6993C-76AA-4486-BAEB-D3828B430A6E/PFOS_En.pdf.]
Environment and Climate Change Canada, 2018, Canadian Environmental Protection Act, 1999—Federal environmental quality guidelines—Perfluorooctane sulfonate (PFOS): Government of Canada, Environment and Climate Change Canada, 14 p. [Also available at https://www.canada.ca/en/environment-climate-change/services/evaluating-existing-substances/federal-environmental-quality-guidelines-perfluorooctane-s ulfonate.html.]
European Chemicals Agency, 2023, Annex I, part A—Priority substances & pollutants in water: European Chemicals Agency database, accessed March 9, 2023, at https://echa.europa.eu/environmental-quality-standards?p_p_id=eucleflegislationlist_WAR_euclefportlet&p_p_lifecycle=1&p_p_state=normal&p_p_mode=view&_ eucleflegislationlist_WAR_euclefportlet_javax.portlet.action=searchLegislationLists.
European Food Safety Authority [EFSA], 2008, EFSA opinion on two environmental pollutants (PFOS and PFOA) present in food: European Food Safety Authority web page, accessed November 12, 2022, at https://www.efsa.europa.eu/en/press/news/080721.
Espartero, L.J.L., Yamada, M., Ford, J., Owens, G., Prow, T., and Juhasz, A., 2022, Health-related toxicity of emerging per- and polyfluoroalkyl substances—Comparison to legacy PFOS and PFOA: Environmental Research, v. 212, p. 113431. [Also available at https://doi.org/10.1016/j.envres.2022.113431.]
Faust, J.A., 2023, PFAS on atmospheric aerosol particles—A review: Environmental Science. Processes & Impacts, v. 25, no. 2, p. 133–150. [Also available at https://doi.org/10.1039/D2EM00002D.]
Felizeter, S., McLachlan, M.S., and De Voogt, P., 2012, Uptake of perfluorinated alkyl acids by hydroponically grown lettuce (Lactuca sativa): Environmental Science & Technology, v. 46, no. 21, p. 11735–11743. [Also available at https://doi.org/10.1021/es302398u.]
Felizeter, S., McLachlan, M.S., and De Voogt, P., 2014, Root uptake and translocation of perfluorinated alkyl acids by three hydroponically grown crops: Journal of Agricultural and Food Chemistry, v. 62, no. 15, p. 3334–3342. [Also available at https://doi.org/10.1021/jf500674j.]
Fiedler, H., Sadia, M., Baabish, A., and Sobhanei, S., 2022, Perfluoroalkane substances in national samples from global monitoring plan projects (2017–2019): Chemosphere, v. 307, p. 136038. [Also available at https://doi.org/10.1016/j.chemosphere.2022.136038.]
Gebreab, K.Y., Benetti, D., Grosell, M., Stieglitz, J.D., and Berry, J.P., 2022, Toxicity of perfluoroalkyl substances (PFAS) toward embryonic stages of mahi-mahi (Coryphaena hippurus): Ecotoxicology (London, England), v. 31, no. 7, p. 1057–1067. [Also available at https://doi.org/10.1007/s10646-022-02576-w.]
Geosyntec Consultants of NC, 2019, Kow, Koc and mass distribution calculations, app. C of Corrective action plan—Chemours Fayetteville Works: The Chemours Company FC, LLC, prepared by Geosyntec Consultants of NC, 8 p., accessed March 8, 2023, at https://files.nc.gov/ncdeq/GenX/consentorder/paragraph-16/FW-CAP-FINAL-12-31-2019-Appendix-C.pdf.
Glaser, D., Lamoureux, E., Opdyke, D., LaRoe, S., Reidy, D., and Connolly, J., 2021, The impact of precursors on aquatic exposure assessment for PFAS—Insights from bioaccumulation modeling: Integrated Environmental Assessment and Management, v. 17, no. 4, p. 705–715. [Also available at https://doi.org/10.1002/ieam.4414.]
Gong, X., Li, K., Wu, C., Wang, L., and Sun, H., 2018, Passive sampling for monitoring polar organic pollutants in water by three typical samplers: Trends in Environmental Analytical Chemistry, v. 17, p. 23–33. [Also available at https://doi.org/10.1016/j.teac.2018.01.002.]
Grasty, R.C., Wolf, D.C., Grey, B.E., Lau, C.S., and Rogers, J.M., 2003, Prenatal window of susceptibility to perfluorooctane sulfonate-induced neonatal mortality in the Sprague-Dawley rat: Birth Defects Research. Part B, Developmental and Reproductive Toxicology, v. 68, no. 6, p. 465–471. [Also available at https://doi.org/10.1002/bdrb.10046.]
Guelfo, J.L., and Higgins, C.P., 2013, Subsurface transport potential of perfluoroalkyl acids at aqueous film-forming foam (AFFF)-impacted sites: Environmental Science & Technology, v. 47, no. 9, p. 4164–4171. [Also available at https://doi.org/10.1021/es3048043.]
Guo, B., Saleem, H., and Brusseau, M.L., 2023, Predicting interfacial tension and adsorption at fluid–fluid interfaces for mixtures of PFAS and/or hydrocarbon surfactants: Environmental Science & Technology, v. 57, no. 21, p. 8044–8052. [Also available at https://doi.org/10.1021/acs.est.2c08601.]
Hammel, E., Webster, T.F., Gurney, R., and Heiger-Bernays, W., 2022, Implications of PFAS definitions using fluorinated pharmaceuticals: iScience, v. 25, no. 4, p. 104020. [Also available at https://doi.org/10.1016/j.isci.2022.104020.]
He, W., Megharaj, M., and Naidu, R., 2016, Toxicity of perfluorooctanoic acid towards earthworm and enzymatic activities in soil: Environmental Monitoring and Assessment, v. 188, no. 7, p. 424. [Also available at https://doi.org/10.1007/s10661-016-5416-y.]
Helmer, R.W., Reeves, D.M., and Cassidy, D.P., 2022, Per- and polyfluorinated alkyl substances (PFAS) cycling within Michigan—Contaminated sites, landfills and wastewater treatment plants: Water Research, v. 210, p. 117983. [Also available at https://doi.org/10.1016/j.watres.2021.117983.]
Higgins, C.P., and Luthy, R.G., 2006, Sorption of perfluorinated surfactants on sediments: Environmental Science & Technology, v. 40, no. 23, p. 7251–7256. [Also available at https://doi.org/10.1021/es061000n.]
Houde, M., De Silva, A.O., Muir, D.C.G., and Letcher, R.J., 2011a, Monitoring of perfluorinated compounds in aquatic biota—An updated review: Environmental Science & Technology, v. 45, no. 19, p. 7962–7973. [Also available at https://doi.org/10.1021/es104326w.]
Houde, M., De Silva, A.O., Muir, D.C.G., and Letcher, R.J., 2011b, Monitoring of perfluorinated compounds in aquatic biota: An updated review: PFCs in aquatic biota: Environmental Science & Technology, v. 45, no. 19, p. 7962–7973. [Also available at https://doi.org/10.1021/es104326w.]
Imbrigiotta, T.E., and Harte, P.T., 2020, Passive sampling of groundwater wells for determination of water chemistry: U.S. Geological Survey Techniques and Methods, chap. 8, book D1, 80 p. [Also available at https://doi.org/10.3133/tm1D8.]
Interstate Technology and Regulatory Council [ITRC], 2021a, Section 7.2 tables ecotoxicology data summary tables: Interstate Technology and Regulatory Council, Excel workbook, accessed March 21, 2023, at https://view.officeapps.live.com/op/view.aspx?src=https%3A%2F%2Fpfas-1.itrcweb.org%2Fwp-content%2Fuploads%2F2022%2F01%2FITRC_PFASSection7.2-TablesEcot oxicologydatasummary-Aug2021.xlsx&wdOrigin=BROWSELINK.
Interstate Technology and Regulatory Council [ITRC],2021b, PFAS technical and regulatory guidance document and fact sheets PFAS–1: Interstate Technology and Regulatory Council web page, accessed March 21, 2023, at https://pfas-1.itrcweb.org/wp-content/uploads/2022/01/PFAS_figure_2-4_family_tree_w_header_120221.pdf.
Interstate Technology and Regulatory Council [ITRC], 2022a, Analytical methods table: Interstate Technology and Regulatory Council web page, accessed March 21, 2023, at https://view.officeapps.live.com/op/view.aspx?src=https%3A%2F%2Fpfas-dev.itrcweb.org%2Fwp-content%2Fuploads%2F2022%2F07%2FITRC_PFASSection11.2_Analyti calMethods_Jun2022-1.xlsx&wdOrigin=BROWSELINK.
Interstate Technology and Regulatory Council [ITRC], 2022b, Biosolids and per- and polyfluoroalkyl substances (PFAS): Interstate Technology and Regulatory Council web page, accessed February 9, 2023, at https://pfas-1.itrcweb.org/wp-content/uploads/2022/10/Biosolids_PFAS_Fact_Sheet_102022_508.pdf.
Interstate Technology and Regulatory Council [ITRC],2022c, PFAS home: Interstate Technology and Regulatory Council web page, accessed February 9, 2023, at https://pfas-1.itrcweb.org/.
Interstate Technology and Regulatory Council [ITRC], 2022d, ITRC PFAS regulatory programs table: Interstate Technology and Regulatory Council web page, accessed March 21, 2023, at https://view.officeapps.live.com/op/view.aspx?src=https%3A%2F%2Fpfas-1.itrcweb.org%2Fwp-content%2Fuploads%2F2022%2F11%2FITRCPFAS_Regulatory_Programs_T able_OCT2022-FINAL.xlsx&wdOrigin=BROWSELINK.
Interstate Technology and Regulatory Council [ITRC], 2022e, ITRC PFAS regulatory programs table: Interstate Technology and Regulatory Council web page, accessed March 21, 2023, at https://pfas-1.itrcweb.org/10-site-characterization/.
Interstate Technology and Regulatory Council [ITRC], 2022f, Water and soil tables of regulations, guidance and advisories for PFAS: Interstate Technology and Regulatory Council web page, accessed March 21, 2023, at https://view.officeapps.live.com/op/view.aspx?src=https%3A%2F%2Fpfas-1.itrcweb.org%2Fwp-content%2Fuploads%2F2022%2F11%2FITRCPFASWaterandSoilValuesTabl es_OCT2022-FINAL.xlsx&wdOrigin=BROWSELINK.
Interstate Technology and Regulatory Council [ITRC],2023, Site characterization: Interstate Technology and Regulatory Council web page, accessed February 9, 2023, at https://pfas-1.itrcweb.org/10-site-characterization/.
Ji, K., Kim, Y., Oh, S., Ahn, B., Jo, H., and Choi, K., 2008, Toxicity of perfluorooctane sulfonic acid and perfluorooctanoic acid on freshwater macroinvertebrates (Daphnia magna and Moina macrocopa) and fish (Oryzias latipes): Environmental Toxicology and Chemistry, v. 27, no. 10, p. 2159–2168. [Also available at https://doi.org/10.1897/07-523.1.]
Kabadi, S.V., Fisher, J., Aungst, J., and Rice, P., 2018, Internal exposure-based pharmacokinetic evaluation of potential for biopersistence of 6:2 fluorotelomer alcohol (FTOH) and its metabolites: Food and Chemical Toxicology, v. 112, p. 375–382. [Also available at https://doi.org/10.1016/j.fct.2018.01.012.]
Karnjanapiboonwong, A., Deb, S.K., Subbiah, S., Wang, D., and Anderson, T.A., 2018, Perfluoroalkylsulfonic and carboxylic acids in earthworms (Eisenia fetida)—Accumulation and effects results from spiked soils at PFAS concentrations bracketing environmental relevance: Chemosphere, v. 199, p. 168–173. [Also available at https://doi.org/10.1016/j.chemosphere.2018.02.027.]
Keeler, B.L., Polasky, S., Brauman, K.A., Johnson, K.A., Finlay, J.C., O’Neill, A., Kovacs, K., and Dalzell, B., 2012, Linking water quality and well-being for improved assessment and valuation of ecosystem services: Proceedings of the National Academy of Sciences of the United States of America, v. 109, no. 45, p. 18619–18624. [Also available at https://doi.org/10.1073/pnas.1215991109.]
Kolanczyk, R.D., Saley, M.R., Serrano, J.A., Daley, S.M., and Tapper, M.A., 2023, PFAS biotransformation pathways—A species comparison study: Toxics, v. 11, no. 1, 24 p. [Also available at https://doi.org/10.3390/toxics11010074.]
Krippner, J., Brunn, H., Falk, S., Georgii, S., Schubert, S., and Stahl, T., 2014, Effects of chain length and pH on the uptake and distribution of perfluoroalkyl substances in maize (Zea mays): Chemosphere, v. 94, p. 85–90. [Also available at https://doi.org/10.1016/j.chemosphere.2013.09.018.]
Krippner, J., Falk, S., Brunn, H., Georgii, S., Schubert, S., and Stahl, T., 2015, Accumulation potentials of perfluoroalkyl carboxylic acids (PFCAs) and perfluoroalkyl sulfonic acids (PFSAs) in maize (Zea mays): Journal of Agricultural and Food Chemistry, v. 63, no. 14, p. 3646–3653. [Also available at https://doi.org/10.1021/acs.jafc.5b00012.]
Laitinen, J.A., Koponen, J., Koikkalainen, J., and Kiviranta, H., 2014, Firefighters’ exposure to perfluoroalkyl acids and 2-butoxyethanol present in firefighting foams: Toxicology Letters, v. 231, no. 2, p. 227–232. [Also available at https://doi.org/10.1016/j.toxlet.2014.09.007.]
Lam, N.H., Cho, C.-R., Kannan, K., and Cho, H.-S., 2017, A nationwide survey of perfluorinated alkyl substances in waters, sediment and biota collected from aquatic environment in Vietnam—Distributions and bioconcentration profiles: Journal of Hazardous Materials, v. 323, p. 116–127. [Also available at https://doi.org/10.1016/j.jhazmat.2016.04.010.]
Langberg, H.A., Hale, S.E., Breedveld, G.D., Jenssen, B.M., and Jartun, M., 2022, A review of PFAS fingerprints in fish from Norwegian freshwater bodies subject to different source inputs: Environmental Science. Processes & Impacts, v. 24, no. 2, p. 330–342. [Also available at https://doi.org/10.1039/D1EM00408E.]
Lath, S., Knight, E.R., Navarro, D.A., Kookana, R.S., and McLaughlin, M.J., 2019, Sorption of PFOA onto different laboratory materials—Filter membranes and centrifuge tubes: Chemosphere, v. 222, p. 671–678. [Also available at https://doi.org/10.1016/j.chemosphere.2019.01.096.]
Lau, C., Anitole, K., Hodes, C., Lai, D., Pfahles-Hutchens, A., and Seed, J., 2007, Perfluoroalkyl acids—A review of monitoring and toxicological findings: Toxicological Sciences, v. 99, no. 2, p. 366–394. [Also available at https://doi.org/10.1093/toxsci/kfm128.]
Lau, C., Thibodeaux, J.R., Hanson, R.G., Narotsky, M.G., Rogers, J.M., Lindstrom, A.B., and Strynar, M.J., 2006, Effects of perfluorooctanoic acid exposure during pregnancy in the mouse: Toxicological Sciences, v. 90, no. 2, p. 510–518. [Also available at https://doi.org/10.1093/toxsci/kfj105.]
Letcher, R.J., Su, G., Moore, J.N., Williams, L.L., Martin, P.A., de Solla, S.R., and Bowerman, W.W., 2015, Perfluorinated sulfonate and carboxylate compounds and precursors in herring gull eggs from across the Laurentian Great Lakes of North America—Temporal and recent spatial comparisons and exposure implications: Science of the Total Environment, v. 538, p. 468–477. [Also available at https://doi.org/10.1016/j.scitotenv.2015.08.083.
Li, F., Fang, X., Zhou, Z., Liao, X., Zou, J., Yuan, B., and Sun, W., 2019, Adsorption of perfluorinated acids onto soils—Kinetics, isotherms, and influences of soil properties: Science of the Total Environment, v. 649, p. 504–514. [Also available at https://doi.org/10.1016/j.scitotenv.2018.08.209.]
Li, L., Zhai, Z., Liu, J., and Hu, J., 2015, Estimating industrial and domestic environmental releases of perfluorooctanoic acid and its salts in China from 2004 to 2012: Chemosphere, v. 129, p. 100–109. [Also available at https://doi.org/10.1016/j.chemosphere.2014.11.049.]
Li, M.-H., 2009, Toxicity of perfluorooctane sulfonate and perfluorooctanoic acid to plants and aquatic invertebrates: Environmental Toxicology, v. 24, no. 1, p. 95–101. [Also available at https://doi.org/10.1002/tox.20396.]
Li, W., Hu, Y., and Bischel, H.N., 2021, In-vitro and in-silico assessment of per- and polyfluoroalkyl substances (PFAS) in aqueous film-forming foam (AFFF) binding to human serum albumin: Toxics, v. 9, no. 3, p. 63. [Also available at https://doi.org/10.3390/toxics9030063.]
Lin, Y., Capozzi, S.L., Lin, L., and Rodenburg, L.A., 2021, Source apportionment of perfluoroalkyl substances in Great Lakes fish: Environmental Pollution, v. 290, p. 118047. [Also available at https://doi.org/10.1016/j.envpol.2021.118047.]
Liu, Y., Zhang, Y., Li, J., Wu, N., Li, W., and Niu, Z., 2019, Distribution, partitioning behavior and positive matrix factorization-based source analysis of legacy and emerging polyfluorinated alkyl substances in the dissolved phase, surface sediment and suspended particulate matter around coastal areas of Bohai Bay, China: Environmental Pollution, v. 246, p. 34–44. [Also available at https://doi.org/10.1016/j.envpol.2018.11.113.]
Lu, G., Liu, J., Sun, L., and Yuan, L., 2015, Toxicity of perfluorononanoic acid and perfluorooctane sulfonate to Daphnia magna: Water Science and Engineering, v. 8, no. 1, p. 40–48. [Also available at https://doi.org/10.1016/j.wse.2015.01.001.]
Luebker, D.J., York, R.G., Hansen, K.J., Moore, J.A., and Butenhoff, J.L., 2005, Neonatal mortality from in utero exposure to perfluorooctanesulfonate (PFOS) in Sprague-Dawley rats—Dose-response, and biochemical and pharamacokinetic parameters: Toxicology, v. 215, no. 1-2, p. 149–169. [Also available at https://doi.org/10.1016/j.tox.2005.07.019.
Lyu, X., Xiao, F., Shen, C., Chen, J., Park, C.M., Sun, Y., Flury, M., and Wang, D., 2022, Per- and polyfluoroalkyl substances (PFAS) in subsurface environments—occurrence, fate, transport, and research prospect: Reviews of Geophysics, v. 60, no. 3, p. e2021RG000765. [Also available at https://doi.org/10.1029/2021RG000765.]
Lyu, Y., Brusseau, M.L., Chen, W., Yan, N., Fu, X., and Lin, X., 2018, Adsorption of PFOA at the air-water interface during transport in unsaturated porous media: Environmental Science & Technology, v. 52, no. 14, p. 7745–7753. [Also available at https://doi.org/10.1021/acs.est.8b02348.]
Mahinroosta, R., and Senevirathna, L., 2020, A review of the emerging treatment technologies for PFAS contaminated soils: Journal of Environmental Management, v. 255, p. 109896. [Also available at https://doi.org/10.1016/j.jenvman.2019.109896.]
Martin, J.W., Mabury, S.A., Solomon, K.R., and Muir, D.C.G., 2003a, Bioconcentration and tissue distribution of perfluorinated acids in rainbow trout (Oncorhynchus mykiss): Environmental Toxicology and Chemistry, v. 22, no. 1, p. 196–204. [Also available at https://doi.org/10.1002/etc.5620220126.]
Martin, J.W., Mabury, S.A., Solomon, K.R., and Muir, D.C.G., 2003b, Bioconcentration and tissue distribution of perfluorinated acids in rainbow trout (Oncorhynchus mykiss): Environmental Toxicology and Chemistry, v. 22, no. 1, p. 196–204. [Also available at https://doi.org/10.1002/etc.5620220126.]
Maine Department of Inland Fisheries and Wildlife [MDIFW], 2022, PFAS in deer harvested in the Fairfield area, Maine—Fall 2021 targeted sampling and advisory summary report: Maine Department of Inland Fisheries and Wildlife report, 18 p. [Also available at https://www.maine.gov/ifw/docs/Maine%20PFOS%20Deer%20Study%20Report%202.8.22_FINAL.pdf.]
Maine State Legislature, 2022, An Act To prevent the further contamination of the soils and waters of the state with so-called forever chemicals: Maine State Legislature Bill LD1911. [Also available at https://www.mainelegislature.org/legis/bills/getPDF.asp?paper=HP1417&item=8&snum=130.]
Michigan Department of Health and Human Services [MIDHHS], 2021, PFAS levels in Michigan Deer from the Oscoda Area, Iosco County: Michigan Department of Health and Human Services report, MDHHS–Pub–1352, 44 p. [Also available at https://www.michigan.gov/-/media/Project/Websites/PFAS-Response/Reports/Report-2021-PFAS-Deer-Oscoda-Area.pdf.]
Mills, M., 2020, PFAS treatment in biosolids—State of the science: U.S. Environmental Protection Agency web page, accessed August 15, 2023, at https://www.epa.gov/research-states/pfas-treatment-biosolids-state-science.
Mommaerts, V., Hagenaars, A., Meyer, J., De Coen, W., Swevers, L., Mosallanejad, H., and Smagghe, G., 2011, Impact of a perfluorinated organic compound PFOS on the terrestrial pollinator Bombus terrestris (Insecta, Hymenoptera): Ecotoxicology (London, England), v. 20, no. 2, p. 447–456. [Also available at https://doi.org/10.1007/s10646-011-0596-2.]
Moody, C.A., and Field, J.A., 1999, Determination of perfluorocarboxylates in groundwater impacted by fire-fighting activity: Environmental Science & Technology, v. 33, no. 16, p. 2800–2806. [Also available at https://doi.org/10.1021/es981355+.]
Moody, C.A., Hebert, G.N., Strauss, S.H., and Field, J.A., 2003, Occurrence and persistence of perfluorooctanesulfonate and other perfluorinated surfactants in groundwater at a fire-training area at Wurtsmith Air Force Base, Michigan, USA: Journal of Environmental Monitoring, v. 5, no. 2, p. 341–345. [Also available at https://doi.org/10.1039/b212497a.]
Morales-McDevitt, M.E., Becanova, J., Blum, A., Bruton, T.A., Vojta, S., Woodward, M., and Lohmann, R., 2021, The air that we breathe—Neutral and volatile PFAS in indoor air: Environmental Science & Technology Letters, v. 8, no. 10, p. 897–902. [Also available at https://doi.org/10.1021/acs.estlett.1c00481.]
Munoz, G., Mercier, L., Duy, S.V., Liu, J., Sauvé, S., and Houde, M., 2022, Bioaccumulation and trophic magnification of emerging and legacy per- and polyfluoroalkyl substances (PFAS) in a St. Lawrence River food web: Environmental Pollution, v. 309, p. 119739. [Also available at https://doi.org/10.1016/j.envpol.2022.119739.]
National Library of Medicine [NIH], 2004, PubChem open chemistry database: NIH webpage accessed March 8, 2023, at https://pubchem.ncbi.nim.nih.gov.
Newsted, J.L., Jones, P.D., Coady, K., and Giesy, J.P., 2005, Avian toxicity reference values for perfluorooctane sulfonate: Environmental Science & Technology, v. 39, no. 23, p. 9357–9362. [Also available at https://doi.org/10.1021/es050989v.]
Ng, C., and Hungerbuehler, K., 2013, Bioconcentration of perfluorinated alkyl acids—How important is specific binding?: Environmental Science & Technology, v. 47, no. 13, p. 7214–7223. [Also available at https://doi.org/10.1021/es400981a.]
Ng, C.A., and Hungerbühler, K., 2014, Bioaccumulation of perfluorinated alkyl acids—Observations and models: Environmental Science & Technology, v. 48, no. 9, p. 4637–4648. [Also available at https://doi.org/10.1021/es404008g.]
Nguyen, T., 2022, Results of EPA’s analytical chemistry branch laboratory study of PFAS leaching from fluorinated HDPE containers—ACB Project B21-02: U.S. Environmental Protection Agency Memorandum, 8 p., accessed November 14, 2022, at https://www.epa.gov/system/files/documents/2022-09/EPA%20PFAS%20Container%20Leaching%20Study%2008122022_0.pdf.
Nian, M., Luo, K., Luo, F., Aimuzi, R., Huo, X., Chen, Q., Tian, Y., and Zhang, J., 2020, Association between prenatal exposure to PFAS and fetal sex hormones—Are the short-chain PFAS safer?: Environmental Science & Technology, v. 54, no. 13, p. 8291–8299. [Also available at https://doi.org/10.1021/acs.est.0c02444.]
Nilsson, H., Kärrman, A., Rotander, A., van Bavel, B., Lindström, G., and Westberg, H., 2013, Biotransformation of fluorotelomer compound to perfluorocarboxylates in humans: Environment International, v. 51, p. 8–12. [Also available at https://doi.org/10.1016/j.envint.2012.09.001.]
Ochoa-Herrera, V., Field, J.A., Luna-Velasco, A., and Sierra-Alvarez, R., 2016, Microbial toxicity and biodegradability of perfluorooctane sulfonate (PFOS) and shorter chain perfluoroalkyl and polyfluoroalkyl substances (PFASs): Environmental Science. Processes & Impacts, v. 18, no. 9, p. 1236–1246. [Also available at https://doi.org/10.1039/C6EM00366D.]
Oun, A., Kumar, A., Harrigan, T., Angelakis, A., and Xagoraraki, I., 2014, Effects of biosolids and manure application on microbial water quality in rural areas in the US: Water (Basel), v. 6, no. 12, p. 3701–3723. [Also available at https://doi.org/10.3390/w6123701.]
Palazzolo, S., Caligiuri, I., Sfriso, A.A., Mauceri, M., Rotondo, R., Campagnol, D., Canzonieri, V., and Rizzolio, F., 2022, Early warnings by liver organoids on short- and long-chain PFAS toxicity: Toxics, v. 10, no. 2, p. 91. [Also available at https://doi.org/10.3390/toxics10020091.]
Pepper, I.L., Brusseau, M.L., Prevatt, F.J., and Escobar, B.A., 2021, Incidence of Pfas in soil following long-term application of class B biosolids: Science of the Total Environment, v. 793, p. 148449. [Also available at https://doi.org/10.1016/j.scitotenv.2021.148449.]
Perkins, R.G., Butenhoff, J.L., Kennedy, G.L., Jr., and Palazzolo, M.J., 2004, 13-week dietary toxicity study of ammonium perfluorooctanoate (APFO) in male rats: Drug and Chemical Toxicology, v. 27, no. 4, p. 361–378. [Also available at https://doi.org/10.1081/DCT-200039773.]
Pfotenhauer, D., Sellers, E., Olson, M., Praedel, K., and Shafer, M., 2022, PFAS concentrations and deposition in precipitation—An intensive 5-month study at National Atmospheric Deposition Program—National trends sites (NADP-NTN) across Wisconsin, USA: Atmospheric Environment, v. 291, p. 119368. [Also available at https://doi.org/10.1016/j.atmosenv.2022.119368.]
Pickard, H.M., Ruyle, B.J., Thackray, C.P., Chovancova, A., Dassuncao, C., Becanova, J., Vojta, S., Lohmann, R., and Sunderland, E.M., 2022, PFAS and precursor bioaccumulation in freshwater recreational fish—Implications for fish advisories: Environmental Science & Technology, v. 56, no. 22, p. 15573–15583. [Also available at https://doi.org/10.1021/acs.est.2c03734.]
Podder, A., Sadmani, A.H.M.A., Reinhart, D., Chang, N.B., and Goel, R., 2021, Per and poly-fluoroalkyl substances (PFAS) as a contaminant of emerging concern in surface water—A transboundary review of their occurrences and toxicity effects: Journal of Hazardous Materials, v. 419, p. 126361. [Also available at https://doi.org/10.1016/j.jhazmat.2021.126361.]
Point, A.D., Holsen, T.M., Fernando, S., Hopke, P.K., and Crimmins, B.S., 2021, Trends (2005–2016) of perfluoroalkyl acids in top predator fish of the Laurentian Great Lakes: Science of the Total Environment, v. 778, p. 146151. [Also available at https://doi.org/10.1016/j.scitotenv.2021.146151.]
Prevedouros, K., Cousins, I.T., Buck, R.C., and Korzeniowski, S.H., 2006, Sources, fate and transport of perfluorocarboxylates: Environmental Science & Technology, v. 40, no. 1, p. 32–44. [Also available at https://doi.org/10.1021/es0512475.]
Princz, J., Jatar, M., Lemieux, H., and Scroggins, R., 2018, Perfluorooctane sulfonate in surface soils—Effects on reproduction in the collembolan, Folsomia candida, and the oribatid mite, Oppia nitens: Chemosphere, v. 208, p. 757–763. [Also available at https://doi.org/10.1016/j.chemosphere.2018.06.020.]
Pronschinske, M.A., Loken, L.C., Corsi, S.R., and Alvarez, D.A., 2023, Per- and polyfluoroalkyl substances (PFAS) and pharmaceutical compound data from passive and sediment samples from 62 Great Lakes tributary sites collected in 2018: U.S. Geological Survey data release, https://doi.org/10.5066/P9QKDUCH.
Qu, B., Zhao, H., and Zhou, J., 2010, Toxic effects of perfluorooctane sulfonate (PFOS) on wheat (Triticum aestivum L.) plant: Chemosphere, v. 79, no. 5, p. 555–560. [Also available at https://doi.org/10.1016/j.chemosphere.2010.02.012.]
Radi, A.B., Noll, K.E., and Oskouie, A.K., 2022, Per- and polyfluoroalkyl substances—Background information with focus on modeling of fate and transport of per- and polyfluroalkyl substances in air media: Journal of Environmental Engineering, v. 148, no. 6, p. 13. [Also available at https://doi.org/10.1061/(ASCE)EE.1943-7870.0001989.]
Rericha, Y., Cao, D.P., Truong, L., Simonich, M.T., Field, J.A., and Tanguay, R.L., 2022, Sulfonamide functional head on short-chain perfluorinated substance drives developmental toxicity: iScience, v. 25, no. 2, p. 15. [Also available at https://doi.org/10.1016/j.isci.2022.103789.]
Rice, P.A., Aungst, J., Cooper, J., Bandele, O., and Kabadi, S.V., 2020, Comparative analysis of the toxicological databases for 6:2 fluorotelomer alcohol (6:2 FTOH) and perfluorohexanoic acid (PFHxA): Food and Chemical Toxicology, v. 138, p. 111210. [Also available at https://doi.org/10.1016/j.fct.2020.111210.]
Rodowa, A.E., Christie, E., Sedlak, J., Peaslee, G.F., Bogdan, D., DiGuiseppi, B., and Field, J.A., 2020, field sampling materials unlikely source of contamination for perfluoroalkyl and polyfluoroalkyl substances in field samples: Environmental Science & Technology Letters, v. 7, no. 3, p. 156–163. [Also available at https://doi.org/10.1021/acs.estlett.0c00036.]
Roth, J., Abusallout, I., Hill, T., Holton, C., Thapa, U., and Hanigan, D., 2020, Release of volatile per- and polyfluoroalkyl substances from aqueous film-forming foam: Environmental Science & Technology Letters, v. 7, no. 3, p. 164–170. [Also available at https://doi.org/10.1021/acs.estlett.0c00052.]
Royal Society of Chemistry [RSC], 2023, ChemSpider: Royal Society of Chemistry database, accessed March 8, 2023, at https://www.chemspider.com/.
Ruffle, B., Vedagiri, U., Bogdan, D., Maier, M., Schwach, C., and Murphy-Hagan, C., 2020, Perfluoroalkyl substances in U.S. market basket fish and shellfish: Environmental Research, v. 190, p. 109932. [Also available at https://doi.org/10.1016/j.envres.2020.109932.]
Ruyle, B.J., Pickard, H.M., LeBlanc, D.R., Tokranov, A.K., Thackray, C.P., Hu, X.C., Vecitis, C.D., and Sunderland, E.M., 2021a, Isolating the AFFF signature in coastal watersheds using oxidizable pfas precursors and unexplained organofluorine: Environmental Science & Technology, v. 55, no. 6, p. 3686–3695. [Also available at https://doi.org/10.1021/acs.est.0c07296.]
Ruyle, B.J., Thackray, C.P., McCord, J.P., Strynar, M.J., Mauge-Lewis, K.A., Fenton, S.E., and Sunderland, E.M., 2021b, Reconstructing the composition of per- and polyfluoroalkyl substances in contemporary aqueous film-forming foams: Environmental Science & Technology Letters, v. 8, no. 1, p. 59–65. [Also available at https://doi.org/10.1021/acs.estlett.0c00798.]
Saini, A., Chinnadurai, S., Schuster, J.K., Eng, A., and Harner, T., 2023, Per- and polyfluoroalkyl substances and volatile methyl siloxanes in global air—And trends: Environmental Pollution, v. 323, p. 121291. [Also available at https://doi.org/10.1016/j.envpol.2023.121291.]
Salvatore, D., Mok, K., Garrett, K.K., Poudrier, G., Brown, P., Birnbaum, L.S., Goldenman, G., Miller, M.F., Patton, S., Poehlein, M., Varshavsky, J., and Cordner, A., 2022, Presumptive contamination—A new approach to PFAS contamination based on likely sources: Environmental Science & Technology Letters, v. 9, no. 11, p. 983–990. [Also available at https://doi.org/10.1021/acs.estlett.2c00502.]
Sant, K.E., Venezia, O.L., Sinno, P.P., and Timme-Laragy, A.R., 2019, Perfluorobutanesulfonic acid disrupts pancreatic organogenesis and regulation of lipid metabolism in the zebrafish, Danio rerio: Toxicological Sciences, v. 167, no. 1, p. 258–268. [Also available at https://doi.org/10.1093/toxsci/kfy237.]
Schaefer, C.E., Lemes, M.C.S., Schwichtenberg, T., and Field, J.A., 2022, Enrichment of poly- and perfluoroalkyl substances (PFAS) in the surface microlayer and foam in synthetic and natural waters: Journal of Hazardous Materials, v. 440, p. 129782. [Also available at https://doi.org/10.1016/j.jhazmat.2022.129782.]
Schwichtenberg, T., Bogdan, D., Carignan, C.C., Reardon, P., Rewerts, J., Wanzek, T., and Field, J.A., 2020, PFAS and dissolved organic carbon enrichment in surface water foams on a northern U.S. freshwater lake: Environmental Science & Technology, v. 54, no. 22, p. 14455–14464. [Also available at https://doi.org/10.1021/acs.est.0c05697.]
SGS, 2018, Physical and chemical properties of PFAS compounds: SGS digital data, accessed March 8, 2023, at https://www.sgs-ehsusa.com/wp-content/uploads/2018/09/Physical-and-Chemical-Properties-of-PFAS-compounds_vKFMH.pdf.
Shi, Y.L., Wang, J.M., Pan, Y.Y., and Cai, Y.Q., 2012, Tissue distribution of perfluorinated compounds in farmed freshwater fish and human exposure by consumption: Environmental Toxicology and Chemistry, v. 31, no. 4, p. 717–723. [Also available at https://doi.org/10.1002/etc.1758.]
Shoemaker, J., and Tettenhorst, D., 2020, Method 537.1 Determination of selected per- and polyflourinated alkyl substances in drinking water by solid phase extraction and liquid chromatography/tandem mass spectrometry (LC/MS/MS) (ver. 2.0): U.S. Environmental Protection Agency report EPA/600/R–20/006, 50 p., accessed July 25, 2023, at https://cfpub.epa.gov/si/si_public_record_report.cfm?Lab=NERL&dirEntryId=348508.
Sims, J.L., Stroski, K.M., Kim, S., Killeen, G., Ehalt, R., Simcik, M.F., and Brooks, B.W., 2022, Global occurrence and probabilistic environmental health hazard assessment of per- and polyfluoroalkyl substances (PFASs) in groundwater and surface waters: Science of the Total Environment, v. 816, p. 151535. [Also available at https://doi.org/10.1016/j.scitotenv.2021.151535.]
Siros, S.M., and Lawson, M.G., 2021, Unexplained PFAS contamination at petroleum spill site mystifies environmental regulators: Jenner & Block web page, accessed March 8, 2023, at https://www.jenner.com/en/news-insights/publications/unexplained-pfas-contamination-at-petroleum-spill-site-mystifies-environmental-regulators.
Soucek, D.J., Dorman, R.A., Pulster, E.L., Perrotta, B.G., Walters, D.M., and Steevens, J.A., 2023, Perfluorooctanesulfonate adversely affects a mayfly (Neocloeon triangulifer) at environmentally realistic concentrations: Environmental Science & Technology Letters, v. 10, no. 3, p. 254–259. [Also available at https://doi.org/10.1021/acs.estlett.3c00056.]
Stahl, L.L., Snyder, B.D., Olsen, A.R., Kincaid, T.M., Wathen, J.B., and McCarty, H.B., 2014, Perfluorinated compounds in fish from U.S. urban rivers and the Great Lakes: Science of the Total Environment, v. 499, p. 185–195. [Also available at https://doi.org/10.1016/j.scitotenv.2014.07.126.]
Sun, J., Letcher, R.J., Eens, M., Covaci, A., and Fernie, K.J., 2020, Perfluoroalkyl acids and sulfonamides and dietary, biological and ecological associations in peregrine falcons from the Laurentian Great Lakes Basin, Canada: Environmental Research, v. 191, p. 110151. [Also available at https://doi.org/10.1016/j.envres.2020.110151.]
Taniyasu, S., Kannan, K., Horii, Y., Hanari, N., and Yamashita, N., 2003, A survey of perfluorooctane sulfonate and related perfluorinated organic compounds in water, fish, birds, and humans from Japan: Environmental Science & Technology, v. 37, no. 12, p. 2634–2639. [Also available at https://doi.org/10.1021/es0303440.]
Taylor, A.C., Fones, G.R., Vrana, B., and Mills, G.A., 2021, Applications for passive sampling of hydrophobic organic contaminants in water—A review: Critical Reviews in Analytical Chemistry, v. 51, no. 1, p. 20–54. [Also available at https://doi.org/10.1080/10408347.2019.1675043.]
Thackray, C.P., Selin, N.E., and Young, C.J., 2020, A global atmospheric chemistry model for the fate and transport of PFCAs and their precursors: Environmental Science. Processes & Impacts, v. 22, no. 2, p. 285–293. [Also available at https://doi.org/10.1039/C9EM00326F.]
The NELAC Institute, 2023, TNI LAMS National Environmental Laboratory Accreditation Management System: The NELAC Institute web page, accessed July 25, 2023, at https://lams.nelac-institute.org/.
Ulhaq, M., Carlsson, G., Örn, S., and Norrgren, L., 2013, Comparison of developmental toxicity of seven perfluoroalkyl acids to zebrafish embryos: Environmental Toxicology and Pharmacology, v. 36, no. 2, p. 423–426. [Also available at https://doi.org/10.1016/j.etap.2013.05.004.]
U.S. Environmental Protection Agency [EPA], 1991, Risk assessment guidance for superfund—Volume 1—Human health evaluation manual (part B, development of risk-based preliminary remediation goals): U.S. Environmental Protection Agency, Office of Emergency and Remedial Response publication 9285.7–01B. [Also available at https://semspub.epa.gov/work/HQ/156739.pdf.]
U.S Environmental Protection Agency [EPA], 1996, Soil Screening Guidance: Fact Sheet: U.S. Environmental Protection Agency, Office of Emergency and Remedial Response publication 9355.4–14FSA, 540–F–95–041 PB96–963501. [Also available at https://semspub.epa.gov/work/HQ/175229.pdf.]
U.S. Environmental Protection Agency [EPA], 2019, Method 533 determination of per- and polyfluoroalkyl substances in drinking water by isotope dilution anion exchange solid phase extraction and liquid chromatography/tandem mass spectrometry: U.S. Environmental Protection Agency report 815–B–19–020, 52 p., accessed July 25, 2023, at https://www.epa.gov/sites/default/files/2019-12/documents/method-533-815b19020.pdf.
U.S. Environmental Protection Agency [EPA], 2002, Supplemental guidance for developing soil screening levels for superfund sites: U.S. Environmental Protection Agency, Solid Waste and Emergency Response publication OSWER 9355–4–24. [Also available at https://semspub.epa.gov/work/HQ/175878.pdf.]
U.S. Environmental Protection Agency [EPA], 2021, Human health toxicity values for hexafluoropropylene oxide (HFPO) dimer acid and its ammonium salt (CASRN 13252-13-6 and CASRN 62037-80-3) also known as “GenX chemicals”: U.S. Environmental Protection Agency document 822R–21–010. [Also available at https://www.epa.gov/system/files/documents/2021-10/genx-chemicals-toxicity-assessment_tech-edited_oct-21-508.pdf.]
U.S. Environmental Protection Agency [EPA], 2022a, Laboratories approved by EPA to support UCMR 5: U.S. Environmental Protection Agency report, 7 p., accessed July 25, 2023, at https://www.epa.gov/dwucmr/list-laboratories-approved-epa-fifth-unregulated-contaminant-monitoring-rule-ucmr-5.
U.S. Environmental Protection Agency [EPA], 2022b, Draft method 1621 screening method for the determination of adsorbable organic fluroine (AOF) in aqueous matrices by combustion ion chromatography (CIC): U.S. Environmental Protection Agency report 821–D–22–002, 36 p., accessed July 25, 2023, at https://www.epa.gov/system/files/documents/2022-04/draft-method-1621-for-screening-aof-in-aqueous-matrices-by-cic_0.pdf.
U.S. Environmental Protection Agency [EPA], 2022c, Draft 2022 aquatic life ambient water quality criteria for perfluorooctanoic acid (PFOA) and perfluorooctane sulfonic acid (PFOS): U.S. Environmental Protection Agency Fact Sheet 842–D–22–005, accessed March 10, 2023, at https://www.epa.gov/ system/files/documents/2022-04/pfoa-pfos-draft-factsheet-2022.pdf.
U.S. Environmental Protection Agency [EPA], 2022d, Lifetime drinking water health advisories for four perfluoroalkyl substances U.S. Code of Federal Register: U.S. Environmental Protection Agency report FRL 9855–01–OW, 2 p., accessed November 3, 2023, at https://www.govinfo.gov/content/pkg/FR-2022-06-21/pdf/2022-13158.pdf.
U.S. Environmental Protection Agency [EPA], 2023a, CompTox Chemicals Dashboard resource hub: U.S. Environmental Protection Agency web page, accessed December 29, 2022, at https://www.epa.gov/comptox-tools/comptox-chemicals-dashboard-resource-hub.
U.S. Environmental Protection Agency [EPA], 2023b, Fact Sheet—2010/2015 PFOA Stewardship Program: U.S. Environmental Protection Agency web page, accessed April 2023 at https://epa.gov/assessing-and-managing-chemicals-under-tsca/fact-sheet-20102015-pfoa-stewardship-program.
U.S Environmental Protection Agency [EPA], 2023c, Risk management for per- and polyfluoroalkyl substances (PFAS) under TSCA: U.S. Environmental Protection Agency web page, accessed February 1, 2023, at https://www.epa.gov/assessing-and-managing-chemicals-under-tsca/risk-management-and-polyfluoroalkyl-substances-pfas.
U.S. Environmental Protection Agency [EPA], 2023d, Understanding the PFAS national primary drinking water proposal hazard index: U.S. Environmental Protection Agency fact sheet, 1 p. [Also available at https://www.epa.gov/system/files/documents/2023-03/How%20do%20I%20calculate%20the%20Hazard%20Index.3.14.23.pdf.]
U.S. Environmental Protection Agency [EPA], 2023e, 2021 TRI national analysis: U.S. Environmental Protection Agency web page, accessed November 30, 2023, at https://www.epa.gov/trinationalanalysis/pfas.
U.S. Environmental Protection Agency [EPA], 2023f, 2022 TRI factsheet—Chemical group—PFAS chemicals (2022 dataset; released October 2023): U.S. Environmental Protection Agency, TRI Explorer web page, accessed November 30, 2023, at https://enviro.epa.gov/triexplorer/tri_factsheet_search.searchfactsheet.
U.S. Environmental Protection Agency [EPA], 2023g, Contaminated site clean-up information (CLU–IN): U.S. Environmental Protection Agency web page, accessed October 23, 2023, at https://clu-in.org/contaminantfocus/default.focus/sec/Per-_and_Polyfluoroalkyl_Substances_(PFAS)/cat/Overview/.
U.S. Environmental Protection Agency [EPA], 2023h, Interim recommendations for addressing groundwater contaminated with PFOA and PFOS: U.S. Environmental Protection Agency web page, accessed June 1, 2023, at https://www.epa.gov/pfas/interim-recommendations-addressing-groundwater-contaminated-pfoa-and-pfos.
U.S. Environmental Protection Agency [EPA], 2023i, PFAS analytical methods development and sampling research: U.S. Environmental Protection Agency web page, accessed April 3, 2023, at https://www.epa.gov/water-research/pfas-analytical-methods-development-and-sampling-research.
U.S. Food and Drug Administration [FDA], 2016, FDA removes approval for the USE of PFCs in food packaging based on the abandonment: U.S. Food and Drug Administration web page, accessed January 11, 2023, at https://www.fda.gov/food/cfsan-constituent-updates/fda-removes-approval-use-pfcs-food-packaging-based-abandonment#:~:text=In%20January%202016%2C%20the %20FDA%20amended%20a%20food,use%20of%20these%20substances%20is%20no%20longer%20allowed.
U.S. Food and Drug Administration [FDA], 2020, FDA announces the voluntary phase-out by industry of certain PFAS used in food packaging: U.S. Food and Drug Administration web page, accessed January 11, 2023, at https://www.fda.gov/food/cfsan-constituent-updates/fda-announces-voluntary-phase-out-industry-certain-pfas-used-food-packaging.
Valsecchi, S., Babut, M., Mazzoni, M., Pascariello, S., Ferrario, C., De Felice, B., Bettinetti, R., Veyrand, B., Marchand, P., and Polesello, S., 2021, Per- and polyfluoroalkyl substances (PFAS) in fish from European lakes—Current contamination status, sources, and perspectives for monitoring: Environmental Toxicology and Chemistry, v. 40, no. 3, p. 658–676. [Also available at https://doi.org/10.1002/etc.4815.]
Van Glubt, S., and Brusseau, M.L., 2021, Contribution of nonaqueous-phase liquids to the retention and transport of per and polyfluoroalkyl substances (PFAS) in porous media: Environmental Science & Technology, v. 55, no. 6, p. 3706–3715. [Also available at https://doi.org/10.1021/acs.est.0c07355.]
Venkatesan, A.K., and Halden, R.U., 2013, National inventory of perfluoroalkyl substances in archived U.S. biosolids from the 2001 EPA National Sewage Sludge Survey: Journal of Hazardous Materials, v. 252–253, p. 413–418. [Also available at https://doi.org/10.1016/j.jhazmat.2013.03.016.]
Vossler, C.A., Dolph, C.L., Finlay, J.C., Keiser, D.A., Kling, C.L., and Phaneuf, D.J., 2023, Valuing improvements in the ecological integrity of local and regional waters using the biological condition gradient: Proceedings of the National Academy of Sciences of the United States of America, v. 120, no. 18, p. e2120251119. [Also available at https://doi.org/10.1073/pnas.2120251119.]
Wallis, D.J., Barton, K.E., Knappe, D.R.U., Kotlarz, N., McDonough, C.A., Higgins, C.P., Hoppin, J.A., and Adgate, J.L., 2023, Source apportionment of serum PFASs in two highly exposed communities: Science of the Total Environment, v. 855, p. 158842. [Also available at https://doi.org/10.1016/j.scitotenv.2022.158842.]
Wang, F., Wang, W., Zhao, D., Liu, J., Lu, P., Rose, N.L., and Zhang, G., 2022, Source apportionment and wet deposition of atmospheric poly- and per-fluoroalkyl substances in a metropolitan city centre of southwest China: Atmospheric Environment, v. 273, p. 118983. [Also available at https://doi.org/10.1016/j.atmosenv.2022.118983.]
Wang, N., Szostek, B., Buck, R.C., Folsom, P.W., Sulecki, L.M., Capka, V., Berti, W.R., and Gannon, J.T., 2005, Fluorotelomer alcohol biodegradation-direct evidence that perfluorinated carbon chains breakdown: Environmental Science & Technology, v. 39, no. 19, p. 7516–7528. [Also available at https://doi.org/10.1021/es0506760.]
Wang, Q., Tsui, M.M.P., Ruan, Y., Lin, H., Zhao, Z., Ku, J.P.H., Sun, H., and Lam, P.K.S., 2019, Occurrence and distribution of per- and polyfluoroalkyl substances (PFASs) in the seawater and sediment of the South China sea coastal region: Chemosphere, v. 231, p. 468–477. [Also available at https://doi.org/10.1016/j.chemosphere.2019.05.162.]
Wang, W., Rhodes, G., Ge, J., Yu, X., and Li, H., 2020, Uptake and accumulation of per- and polyfluoroalkyl substances in plants: Chemosphere, v. 261, p. 127584. [Also available at https://doi.org/10.1016/j.chemosphere.2020.127584.]
Wang, Z., Buser, A.M., Cousins, I.T., Demattio, S., Drost, W., Johansson, O., Ohno, K., Patlewicz, G., Richard, A.M., Walker, G.W., White, G.S., and Leinala, E., 2021, A new OECD definition for per- and polyfluoroalkyl substances: Environmental Science & Technology, v. 55, no. 23, p. 15575–15578. [Also available at https://doi.org/10.1021/acs.est.1c06896.]
Wang, Z., Cousins, I.T., Scheringer, M., Buck, R.C., and Hungerbühler, K., 2014, Global emission inventories for C4–C14 perfluoroalkyl carboxylic acid (PFCA) homologues from 1951 to 2030, Part I—Production and emissions from quantifiable sources: Environment International, v. 70, p. 62–75. [Also available at https://doi.org/10.1016/j.envint.2014.04.013.]
Wang, Z., DeWitt, J.C., Higgins, C.P., and Cousins, I.T., 2017, A never-ending story of per- and polyfluoroalkyl substances (PFASs)?: Environmental Science & Technology, v. 51, no. 5, p. 2508–2518. [Also available at https://doi.org/10.1021/acs.est.6b04806.]
Wen, B., Wu, Y., Zhang, H., Liu, Y., Hu, X., Huang, H., and Zhang, S., 2016, The roles of protein and lipid in the accumulation and distribution of perfluorooctane sulfonate (PFOS) and perfluorooctanoate (PFOA) in plants grown in biosolids-amended soils: Environmental Pollution, v. 216, p. 682–688. [Also available at https://doi.org/10.1016/j.envpol.2016.06.032.]
Wolf, C.J., Fenton, S.E., Schmid, J.E., Calafat, A.M., Kuklenyik, Z., Bryant, X.A., Thibodeaux, J., Das, K.P., White, S.S., Lau, C.S., and Abbott, B.D., 2007, Developmental toxicity of perfluorooctanoic acid in the CD-1 mouse after cross-foster and restricted gestational exposures: Toxicological Sciences, v. 95, no. 2, p. 462–473. [Also available at https://doi.org/10.1093/toxsci/kfl159.]
Wood, C., Balazs, G.H., Rice, M., Work, T.M., Jones, T.T., Sterling, E., Summers, T.M., Brooker, J., Kurpita, L., King, C.S., and Lynch, J.M., 2021, Sea turtles across the North Pacific are exposed to perfluoroalkyl substances: Environmental Pollution, v. 279, p. 116875. [Also available at https://doi.org/10.1016/j.envpol.2021.116875.]
Wu, Y., Simon, K.L., Best, D.A., Bowerman, W., and Venier, M., 2020, Novel and legacy per- and polyfluoroalkyl substances in bald eagle eggs from the Great Lakes region: Environmental Pollution, v. 260, p. 113811. [Also available at https://doi.org/10.1016/j.envpol.2019.113811.]
Xu, D.M., Li, C.D., Wen, Y.Z., and Liu, W.P., 2013, Antioxidant defense system responses and DNA damage of earthworms exposed to perfluorooctane sulfonate (PFOS): Environmental Pollution, v. 174, p. 121–127. [Also available at https://doi.org/10.1016/j.envpol.2012.10.030.]
Zafeiraki, E., Costopoulou, D., Vassiliadou, I., Bakeas, E., and Leondiadis, L., 2014, Determination of perfluorinated compounds (PFCs) in various foodstuff packaging materials used in the Greek market: Chemosphere, v. 94, p. 169–176. [Also available at https://doi.org/10.1016/j.chemosphere.2013.09.092.]
Zareitalabad, P., Siemens, J., Wichern, F., Amelung, W., and Joergensen, R.G., 2013, Dose-dependent reactions of Aporrectodea caliginosa to perfluorooctanoic acid and perfluorooctanesulfonic acid in soil: Ecotoxicology and Environmental Safety, v. 95, p. 39–43. [Also available at https://doi.org/10.1016/j.ecoenv.2013.05.012.]
Zhang, W., Zhang, Y., Zhang, H., Wang, J., Cui, R., and Dai, J., 2012, Sex differences in transcriptional expression of FABPs in zebrafish liver after chronic perfluorononanoic acid exposure: Environmental Science & Technology, v. 46, no. 9, p. 5175–5182. [Also available at https://doi.org/10.1021/es300147w.]
Zhao, H., Chen, C., Zhang, X., Chen, J., and Quan, X., 2011, Phytotoxicity of PFOS and PFOA to Brassica chinensis in different Chinese soils: Ecotoxicology and Environmental Safety, v. 74, no. 5, p. 1343–1347. [Also available at https://doi.org/10.1016/j.ecoenv.2011.03.007.]
Zhao, H., Qu, B., Guan, Y., Jiang, J., and Chen, X., 2016, Influence of salinity and temperature on uptake of perfluorinated carboxylic acids (PFCAs) by hydroponically grown wheat (Triticum aestivum L.): SpringerPlus, v. 5, no. 1, p. 541. [Also available at https://doi.org/10.1186/s40064-016-2016-9.]
Zhao, S., Fang, S., Zhu, L., Liu, L., Liu, Z., and Zhang, Y., 2014, Mutual impacts of wheat (Triticum aestivum L.) and earthworms (Eisenia fetida) on the bioavailability of perfluoroalkyl substances (PFASs) in soil: Environmental Pollution, v. 184, p. 495–501. [Also available at https://doi.org/10.1016/j.envpol.2013.09.032.]
Zhong, H., Zheng, M., Liang, Y., Wang, Y., Gao, W., Wang, Y., and Jiang, G., 2021, Legacy and emerging per- and polyfluoroalkyl substances (PFAS) in sediments from the East China Sea and the Yellow Sea—Occurrence, source apportionment and environmental risk assessment: Chemosphere, v. 282, p. 131042. [Also available at https://doi.org/10.1016/j.chemosphere.2021.131042.]
Zhou, J., Baumann, K., Mead, R.N., Skrabal, S.A., Kieber, R.J., Avery, G.B., Shimizu, M., DeWitt, J.C., Sun, M., Vance, S.A., Bodnar, W., Zhang, Z., Collins, L.B., Surratt, J.D., and Turpin, B.J., 2021, PFOS dominates PFAS composition in ambient fine particulate matter (PM2.5) collected across North Carolina nearly 20 years after the end of its US production: Environmental Science. Processes & Impacts, v. 23, no. 4, p. 580–587. [Also available at https://doi.org/10.1039/D0EM00497A.]
Zhu, W., Khan, K., Roakes, H., Maker, E., Underwood, K.L., Zemba, S., and Badireddy, A.R., 2022, Vermont-wide assessment of anthropogenic background concentrations of perfluoroalkyl substances in surface soils: Journal of Hazardous Materials, v. 438, p. 129479. [Also available at https://doi.org/10.1016/j.jhazmat.2022.129479.]
Zodrow, J.M., Frenchmeyer, M., Dally, K., Osborn, E., Anderson, P., and Divine, C., 2021, Development of per and polyfluoroalkyl substances ecological risk-based screening levels: Environmental Toxicology and Chemistry, v. 40, no. 3, p. 921–936. [Also available at https://doi.org/10.1002/etc.4975.]
Supplemental Information
Concentrations are given in the following units of measure: milligrams per liter (mg/L), nanograms per liter (ng/L), grams per liter (g/L), picograms per cubic meter (pg/m3), micrograms per kilogram (µg/kg), milligrams per kilogram (mg/kg), nanograms per gram (ng/g), parts per million (ppm), parts per billion (ppb), and parts per trillion (pptr).
Abbreviations
~
about
AFFF
aqueous film forming foam
AOF
absorbable organic fluorine
ASTM
American Society for Testing and Materials
AWI
air-water interface
C
number of carbons on the carbon-fluorine backbone
CERCLA
Comprehensive Environmental Response, Compensation, and Liability Act
DoD
U.S. Department of Defense
EPA
U.S. Environmental Protection Agency
EQS
environmental quality standards
FDA
U.S. Food and Drug Administration
FTOH
Fluorotelomer alcohol
ISO
International Organization for Standardization
ITRC
Interstate Technology Regulatory Council
LC/MS/MS
liquid chromatography tandem mass spectrometry
LC–PFCA
long-chain PFCA
MCL
maximum contaminant levels
MCLG
maximum contaminant level goals
MDL
method detection limit
ND
nondetect
NRDAR
Natural Resource Damage Assessment and Restoration
NWI
nonaqueous phase liquid-water interface
PFAA
perfluoroalkyl acids
PFAS
per- and polyfluoroalkyl substances (see table 1 for individual PFAS abbreviations)
PFCA
perfluoroalkyl carboxylic acids/perfluoroalkyl carboxylates
PFSA
perfluoroalkane sulfonic acids/perfluoroalkane sulfonates
PSD
passive sampling devices
PTFE
polytetrafluoroethylene
QC
quality control
RBSL
risk-based screening levels
TOF
total and organic fluorine
TOP
total oxidizable precursor assay
w.w.
wet weight
WWTP
wastewater treatment plant
For more information about this publication, contact:
Director, USGS Columbia Environmental Research Center
4200 New Haven Road
Columbia, MO 65201
573–875–5399
For additional information, visit: https://www.usgs.gov/centers/cerc
Publishing support provided by the Rolla Publishing Service Center
Disclaimers
Any use of trade, firm, or product names is for descriptive purposes only and does not imply endorsement by the U.S. Government.
Although this information product, for the most part, is in the public domain, it also may contain copyrighted materials as noted in the text. Permission to reproduce copyrighted items must be secured from the copyright owner.
Suggested Citation
Pulster, E.L., Bowman, S.R., Keele, L., and Steevens, J., 2024, Guide to per- and polyfluoroalkyl substances (PFAS) sampling within Natural Resource Damage Assessment and Restoration: U.S. Geological Survey Open-File Report 2024–1001, 57 p., https://doi.org/10.3133/ofr20241001.
ISSN: 2331-1258 (online)
Publication type | Report |
---|---|
Publication Subtype | USGS Numbered Series |
Title | Guide to per- and polyfluoroalkyl substances (PFAS) sampling within Natural Resource Damage Assessment and Restoration |
Series title | Open-File Report |
Series number | 2024-1001 |
DOI | 10.3133/ofr20241001 |
Year Published | 2024 |
Language | English |
Publisher | U.S. Geological Survey |
Publisher location | Reston, VA |
Contributing office(s) | Columbia Environmental Research Center |
Description | vi, 57 p. |
Online Only (Y/N) | Y |
Additional Online Files (Y/N) | N |
Google Analytic Metrics | Metrics page |