A Novel Tool to Selectively Deliver a Control Agent to Filter-Feeding Silver and Bighead Carp
Links
- Document: Report (1.0 MB pdf) , HTML , XML
- Data Release: UGSS data release - Data release for a novel tool to selectively deliver a control agent to filter-feeding silver and bighead carp
- Download citation as: RIS | Dublin Core
Acknowledgments
This study was funded by the U.S. Geological Survey Ecosystem Mission Area, Biological Threats and Invasive Species Research Program. We thank Jon Amberg for conceptualization and planning of the study, along with several other team members from the U.S. Geological Survey for participation in various stages of planning, setup, and completion of this study.
Abstract
Invasive carp pose substantial economic and ecological damage when populations are widespread in freshwater systems within the United States. Resource managers in the United States have few chemical control tools to selectively remove nuisance fish. This study examined whether Antimycin–A (antimycin) wax encapsulated microparticles could cause selective lethality in invasive carps. The antimycin microparticles were selective toward bighead carp (Hypophthalmichthys nobilis) and silver carp (Hypophthalmichthys molitrix) across multiple experimental scales. Microparticles applied in experimental pond studies caused approximately 50 percent lethality in invasive carp. Effluent pond studies performed at Rathbun Fish Hatchery (Moravia, Iowa) caused silver carp lethality at a lower rate than previous pond or laboratory studies (approximately 1 percent); however, minimal effects on other fish species were observed. The antimycin microparticle formulation shows the ability to cause lethality in filter-feeding invasive carp relative to other fish species and demonstrated the plausibility for delivering a typically nonselective toxicant in a selective manner to specific species based on their physiological feeding traits.
Introduction
Lethal control agents are an important component to fisheries management. Only four piscicides are registered with the U.S. Environmental Protection Agency (EPA), including 4-nitro-3-(trifluoromethyl)phenol (TFM-HP Sea Lamprey Larvicide; EPA regulation number 6704–45; U.S. Environmental Protection Agency, 1982), niclosamide (Bayluscide Technical; EPA regulation number 6704–88; U.S. Environmental Protection Agency, 2019a), Carbon Dioxide-Carp (EPA regulation number 6704–95; U.S. Environmental Protection Agency, 2019b), and rotenone (Prenfish Fish Toxicant; EPA regulation number 89459–85; U.S. Environmental Protection Agency, 2016). The pesticides 4-nitro-3-(trifluoromethyl)phenol and niclosamide have a restricted-use label, rotenone is labeled as a broad use pesticide, and Carbon Dioxide-Carp is labeled for deterrence and under-ice removal of nuisance species (Fredricks and others, 2021). Another chemical control agent, Antimycin–A (antimycin; Fintrol; EPA regulation number 39096–2; U.S. Environmental Protection Agency, 2012), was nationally registered as a pesticide from 1960 to 2017. Similar to rotenone, antimycin is a nonselective pesticide that was historically used to remove nuisance fish, and 1,138 units of Fintrol were used in the United States between 1991 and 2000 (approximately 50.5 kilograms [kg] of antimycin total over 9 years; Finlayson and others, 2002).
Currently, resource managers only have rotenone and carbon dioxide to control undesirable bony fishes, such as silver carp (Hypophthalmichthys molitrix) and bighead carp (Hypophthalmichthys nobilis), collectively referred to as “invasive carp” in this report. Both species were introduced into the United States to control plankton in effluent ponds (Kolar and others, 2005; Conover and others, 2007). They eventually escaped and have become established throughout multiple river basins in the Central United States (Schrank and Guy, 2002; Conover and others, 2007; DeGrandchamp and others, 2008; Sampson and others, 2009; Coulter and others, 2013). Once established, invasive carp compete with native fish for resources such as food and habitat (Sass and others, 2014). The high primary productivity of midwestern rivers allows invasive carp populations to thrive, and recent estimates indicate their population density in the La Grange reach of the Illinois River to be near the highest in the world (Sass and others, 2010). Invasive carp are highly fecund with relatively fast growth rates, which creates concern for their potential to negatively affect aquatic ecosystems. Silver carp diet overlaps with diets of gizzard shad (Dorosoma cepedianum) and buffalo fishes (genus Ictiobus; Sampson and others, 2009), and they are expected to compete with juvenile sport and commercial fish species within the Great Lakes basin (Cudmore and others, 2012). Silver carp have continued to compete with gizzard shad and bigmouth buffalo (Ictiobus cyprinellus), and as a result, a decline in body condition and catch per unit effort of native planktivores has been noted in the La Grange reach of the Illinois River (Pendleton and others, 2017).
Lethal control agents with species selectivity are of great interest to fisheries managers. The selectivity of control agents may be engineered by exploiting the physiology or behavior of each species of interest. One selectivity strategy under evaluation aims to exploit invasive carp’s filter-feeding behavior, which minimally overlaps with nontarget fish species, by using an oral delivery platform (ODP). Silver carp diets overlap with gizzard shad diets, but there are key differences in the gill rakers of these two species. The size of pores in silver carp gill rakers (95-percent confidence intervals ranged from 80.69 to 185.75 micrometers [μm]) are much larger than the interraker spacings in gizzard shad gill rakers (95-percent confidence intervals ranged from 16.72 to 47.36 μm; Walleser and others, 2014). This size difference allows silver carp to filter a wider range of particle sizes than gizzard shad. Several ODPs have been developed using both rotenone and antimycin previously (Rach and others, 1994; Bonneau and Scarnecchia, 2001). These ODPs were made by embedding bait directly with pesticides but without protecting against the control agent hydrolysis and dissipation. Rotenone baits have limited oral toxicity and palatability (Mallison and others, 1995; Bonneau and Scarnecchia, 2001). More recently, an ODP was created with an encapsulated formulation of antimycin inside a bait material (Poole and others, 2018). Poole and others (2018) observed mortality to common carp (Cyprinus carpio) while minimizing lethality to yellow perch (Perca flavescens) and bluegill (Lepomis macrochirus) during mixed-species exposures; however, high mortality was observed in fathead minnow (Pimephales promelas). Poole and others (2018) hypothesized that bait manufacture modifications could prevent small cyprinids from consuming the toxic bait. These results indicate that mortality was a result of toxic bait consumption dietarily rather than gill uptake and a corresponding antimycin toxicity from the water.
Microencapsulation has been extensively used in the pharmaceutical and food industries and could be useful to deliver lethal control agents to invasive species (Gaonkar and others, 2014). In aquaculture, microencapsulation has been used to deliver salmonid vaccines (Tobar and others, 2011; Caruffo and others, 2016) and nutrients to invertebrates (Langdon and others, 2008; Hawkyard and others, 2014). These microencapsulation technologies have a subset of considerations to make their delivery and target optimal, such as the particle size, core material, physiochemical properties, and control of chemical release mechanisms. Formulation development often includes understanding life history, feeding strategies, feeding stimulants, and digestive physiology. Many of these elements may need to be considered with the microencapsulation of lethal agents for invasive species control, with special emphasis on the palatability and leaching of the control agent from ODPs (Bonneau and Scarnecchia, 2001). Leaching of the control agent can lead to nontarget species lethality if encapsulation is not used to reduce the rate of environmental release (Taverna and others, 2018; Wang and others, 2020).
The U.S. Geological Survey has evaluated different microparticles to identify a formulation that does not leach the chemical control agent, is palatable, and is scalable for commercial production (Kolar and Morrison, 2016; Gaikowski, 2018). The study objectives were to (1) determine the acute toxicity of the different antimycin microparticle formulations exposed to invasive carp and non-target native fish in the laboratory and experimental ponds, (2) determine the leaching rate of antimycin microparticle in water, and (3) examine whether the microparticle delivery system establishes a toxicological selectivity for invasive carp across multiple experimental scales.
Materials and Methods
Antimycin microparticles were evaluated to determine their effectiveness on fish species in a series of laboratory trials, experimental pond trials, and Rathbun Fish Hatchery effluent pond trials. The antimycin microparticle formulation was also evaluated for antimycin leaching.
Test Animals
These studies have been examined by the U.S. Geological Survey Upper Midwest Environmental Sciences Center Animal Care and Use Committee for consistency with the Animal Welfare Act (7 U.S.C. 2131 et seq.) and with rules governing the use of test animals at the U.S. Geological Survey.
Laboratory study test fish were bluegill, largemouth bass (Micropterus salmoides), silver carp, and bighead carp (table 1). Both indigenous (bluegill and largemouth bass) and nonindigenous fish (silver carp) were obtained from the fish culture facility at the U.S. Geological Survey Upper Midwest Environmental Sciences Center. Bighead carp were acquired from the Osage Catfisheries, Inc., in Osage Beach, Missouri. All fish were juveniles (1–3 years old) at the time of each study.
Table 1.
Mean (plus or minus standard deviation) length and mass of each fish species used in Antimycin–A microparticle laboratory trials, experimental pond trials, and Rathbun Fish Hatchery field trials. Data summarized from Sauey and others, 2024.[n, number of fish per experimental chamber; mm, millimeter; g, gram; NA, not available]
Pond study test fishes were bighead carp, silver carp, largemouth bass, bluegill, and grass carp (Ctenopharyngodon idella; table 1). Juvenile bighead carp, silver carp, and grass carp utilized in studies performed at U.S. Geological Survey Columbia Environmental Research Center and fish were collected from the field in the Missouri River near Hartsburg, Mo. (river mile 159.3) via dip netting. Wild fishes were transferred into floating cages placed within 0.1-hectare pond (897,900 liters [L]) earthen ponds. Fish in ponds had access to natural biota, supplemented with daily rations of commercial fish food from Zeigler Bros., Inc., Gardners, Pennsylvania, and approximately 50 grams (g) of a 50/50 algal mixture of dry Chlorella and Spirulina powder.
The fish assemblage in the Rathbun Fish Hatchery effluent pond study consisted primarily of bighead carp, silver carp, bigmouth buffalo (Ictiobus cyprinellus), smallmouth buffalo (Ictiobus bubalus), river carpsucker (Carpiodes carpio), and common carp, all of which were unintendedly introduced during flood events. Other, sparse species previously observed include gizzard shad, channel catfish (Ictalurus punctatus), white bass (Morone chrysops), white crappie (Pomoxis annularis), black crappie (Pomoxis nigromaculatus), bluegill, walleye (Sander vitreus), sauger (Sander canadensis), and green sunfish (Lepomis cyanellus). Before the microparticle laboratory trial, a mark-recapture fish abundance survey was conducted during a 2-week period using hoop nets. Abundance was estimated using the Schumacher-Eschmeyer method (Krebs, 1989).
Microparticle Production
Antimycin microparticles were produced via air atomization similarly to the methods described in Hawkyard and others (2011) and Langdon and others (2008). Briefly, molten wax is sprayed through an air-atomizing spray nozzle into a collection vessel, which has been chilled with liquid nitrogen to solidify the wax microparticles instantaneously. Antimycin (Aquabiotics, Incorporated, Bainbridge Island, Washington) was emulsified in a molten mixture of refined wax at 120 degrees Celsius (°C) and then sprayed using an air atomizing sprayer (Spraying Systems Co., Wheaton, Illinois) with a 35-µm spraying nozzle. The nominal antimycin percentage in the wax was approximately 20 percent weight per weight (hereafter referred to as “type 1”).
A second microparticle was manufactured by top-coating an aliquot of type 1 with an additional layer of wax using the methods described above but with a 60-µm spraying nozzle. Preliminary studies indicated the percentage of antimycin decreased following the second spraying method to approximately 4 percent weight per weight (hereafter referred to as “type 2”). Microparticles used in the Rathbun Fish Hatchery effluent pond study also contained 0.1 percent (weight per weight) yttrium as a dietary tracer to verify fish microparticle consumption (Jobling and others, 2001). All microparticles were immediately frozen after production and stored at −20 °C for later use.
Laboratory Toxicity Studies
Fish were exposed to a type 2 antimycin microparticle in two independent laboratory toxicity studies (trials 1 and 2). Experiments were conducted in 12 cylindrical, flat-bottom, 227-L experimental chambers (81-centimeters high by 61-centimeters diameter tanks) filled with 150 L of well water in a flow-through system at approximately 20 °C. The well water flow rate was set to be 2.5 liters per minute. Each study was conducted with 10 silver carp, bighead carp, largemouth bass, and bluegill in each tank (table 1). Fish were acclimated to test conditions and fasting for 48 hours before testing. After acclimation, duplicate tanks of experimental concentration were dosed with type 2 antimycin microparticles. The water flow was interrupted for 1 hour upon microparticle addition to minimize the loss of the microparticles in the waste effluent. Microparticles were added via hand broadcast to the surface of each tank. Experimental concentrations were determined by the nominal mass of antimycin per total fish weight (milligrams per kilograms total fish) in each tank. The nominal mean (plus or minus [±] standard deviation [SD]) concentrations used in the study were 0.00 (control), 0.20 (0.02), 0.49 (0.03), 0.93 (0.07), and 1.89 (0.19). These concentrations resulted from adding approximately 0.0, 0.1, 0.25, 0.5, and 1.0 g of type 2 microparticles in each tank. Water quality parameters (dissolved oxygen, temperature, and pH; table 2) were measured at 1, 24, and 48 hours after application with a handheld YSI dissolved oxygen and Beckman Coulter Phi 410 pH meter. Fish mortality was assessed every hour for the first 6 hours and then 24 and 48 hours after application. Dead fish were removed at each time point, and standard length (millimeters) and wet-weight (grams) measurements were recorded. Fish remaining at the conclusion of each study were euthanized with an overdose of 200 milligrams per liter (mg/L) Tricaine-S for 15 minutes. The lethal concentration or dose to cause lethality in 50 percent of fish ([LD50]) was calculated using the mean nominal concentration of antimycin (milligrams per kilogram of total fish) with the EPA Toxicity Relationship Analysis Program (v1.30a; Erickson, 2010). Toxicity values and confidence intervals were calculated for each time point (6, 24, and 48 hours) (table 3).
Table 2.
Mean (plus or minus standard deviation) water chemistry parameters of exposure water used in laboratory, pond, and Rathbun Fish Hatchery effluent pond studies of Antimycin–A microparticle. Data summarized from Sauey and others (2024).[n, number; mg/L, milligram per liter; °C, degree Celsius; NA, data not available]
Table 3.
Mean (plus or minus standard deviation; experimental chamber replicate=4, number of fish per experimental chamber=39–41) percent survival of fish species exposed to type 2 Antimycin–A microparticle for 6 hours, 24 hours, and 48 hours. All four fish species (n=9–11 fish per tank) including silver carp, bighead carp, bluegill, and largemouth bass were present in each experimental chamber. Data summarized from Sauey and others (2024).[mg/kg, milligram per kilogram]
Laboratory Dissipation Study
Dissipation studies were conducted with antimycin microparticle type 2 to determine the rate of chemical dissipation in water. Dissipation is the process of the chemical leaching from the wax microparticle into water, chemical degradation, and chemical partitioning elsewhere. The dissipation rate is the measure of chemical dissipation over time. Type 2 antimycin microparticle (for example, 100 milligrams [mg]) contained in a 35-micron nylon mesh bag were added to triplicate glass bottles with 25 milliliters (mL) of ultrapure water (18.3 mega ohms per centimeter squared; Barnstead E-Pure Water System, Thermo Fisher, Waltham, Massachusetts). Triplicate water samples (1 mL) were collected temporally at 30 minutes and 60 minutes in ultrapure water and were processed using Solid Phase Extraction (SPE; Oasis MAX 60 mg, Waters Corp., Milford, Mass.). Additionally, a dissipation study was conducted with ultrapure water and natural river water collected from the Black River in La Crosse, Wisc. The water quality parameters of Black River were characterized using the Long Term Resource Monitoring Program database (table 2; U.S. Army Corps of Engineers and others, 2023). Antimycin microparticle (100 mg) was added to glass bottles in triplicate containing 1.0-L water and stored in the dark at room temperature (20 °C). Triplicate ultrapure water samples, each 2.0 mL, were collected on 1, 3, 7, 10, 14, 18, 22, 26, 30, 33, and 38 days following the addition of microparticle at each time point. Water samples, each 2.0 mL, were collected from the Black River to quantify antimycin occurrence on 1, 3, 7, 10, 13, and 16 days. All water samples were processed using SPE cartridges, as mentioned above, to concentrate antimycin for analytical verification using an Agilent 6530 Accurate-Mass quantitative time-of-flight liquid chromatography/mass spectrometry system, Agilent Technologies, Santa Clara, Calif.) or an Agilent 6460 Triple Quad liquid chromatography/mass spectrometry system according to previous published methods (Bernardy and others, 2013). Instrument configuration and acquisition conditions are summarized in appendix 1. Antimycin (Sigma-Aldrich, St. Louis, Mo.) was dissolved in acetonitrile with 1-percent formic acid to create analytical standards (4.1–256.1 micrograms per liter [µg/L]) for a calibration curve. Scatter plots were created to graph the antimycin water concentration (y-axis) across time (days) and subsequently determine the dissipation rate for each water type. A natural log transformation of the antimycin concentration was necessary to increase linearity. Linear regression analysis was performed to determine whether the slope of each curve was different than zero and calculate the dissipation rate (slope). Significance was declared at probability value (p-value) less than (<) −0.05.
Experimental Pond Study
Two initial proof-of-concept pond trials were conducted to test the microparticle effectiveness on a larger scale. Both trials 1 and 2 pond studies were conducted in a 0.1-hectare pond (897,900 L) at the U.S. Geological Survey Columbia Environmental Research Center from June 1, 2016, to August 11, 2016. A single pond was divided into two using a weighted 1/4-inch mesh seine net (fig. 1) to serve as control and treatment experimental units. Each side of the pond contained a ring constructed of 8-inch polyvinyl chloride (PVC) pipe, with an internal area of 0.75 square meter, to contain an algal attractant and microparticle. Trial 1 contained silver carp (n=20 per side), bighead carp (n=20 per side), and largemouth bass (side A: n=10; side B: n=9). Trial 2 contained silver carp (n=20), bighead carp (n=20), largemouth bass (n=5), and grass carp (n=5) on each side of the pond. The length and weight of fish used in both trials are in table 1. Fish were acclimated to feeding rings in each trial for greater than or equal to7 days before study initiation (Trial 1: 9 days; Trial 2: 48 days). During acclimation, carp were fed approximately 64 g of a 50/50 mixture of Spirulina and Chlorella powder in the feeding rings via telescopic pole, two times per day (morning and afternoon). Water quality parameters (pH, dissolved oxygen, and temperature; table 2) were measured before microparticle addition using a handheld YSI multiparameter water quality meter.

Experimental pond setup at the U.S. Geological Survey.
Type 2 antimycin microparticle (Trial 1: 0.615 g antimycin or 15.80 mg/kg total fish; Trial 2: 0.332 g antimycin or 21.08 mg/kg total fish) was dosed in the morning feeding on the treatment side of the pond and an equal amount of blank microparticle was added to the control side of the pond. Mortality observations were performed at 6 and 24 hours after addition of microparticle, and dead fish were removed and measured for standard length (millimeters) and weight (grams). After 24 hours, the pond was drained, and the remaining fish were counted to record survival. A Fisher’s Exact Test (SigmaPlot v14.5; Systat, Inc.) was performed to determine whether significant (p-value<0.05) lethality occurred between control (experimental replicates [N=2]) versus the microparticle treatment (N=2).
Rathbun Fish Hatchery Effluent Pond Field Study
A semi-controlled field study was conducted to examine the type 1 antimycin microparticle effectiveness on captive wild fish at the Rathbun Fish Hatchery, near Moravia, Iowa. Type 1 microparticle was selected for this study, as opposed to type 2, to increase the dosage of antimycin delivered because the invasive carp were larger than the fish used in previous studies. The effluent discharge system consisted of two 0.81-hectare ponds (6,784,000 L) with a mean water depth of 1.5 meters. Effluent baffles placed at the end of each pond to increase water retention and a containment boom (Super Stream Boom, Elastec, Inc., Carmi, Ill.) placed at the pond outflow were used to prevent microparticle release into the river.
At 6 equidistant points around the pond, feeding rings were placed to apply a liquid algal attractant (50/50 of Spirulina and Chlorella) and subsequent microparticles. Feeding rings were constructed of extruded plastic and closed cell foam (1.5-meters diameter) and held in place by tethering to a cinderblock. A secondary (3-meters diameter) containment ring was constructed and placed around the primary feeding ring to further contain the microparticle. Fish were feed trained by autonomously pumping the liquid algal mixture into feeding rings (approximately 4 L of liquid algal mixture per feeding ring) at 22:00 hours for 14 days before microparticle application, using a Turbo Rain irrigation system attached to a 378-L storage tank (Garden Green EcoSolutions/Turborain, Downers Grove, Ill.).
Following feed training, type 1 antimycin microparticle was added to each feeding ring (approximately 1.016 g of antimycin per feeding ring; approximately 6.095 g of total antimycin in the pond; 0.903 µg/L of total nominal antimycin concentration applied to the pond) with the algal attractant. To ensure the remaining microparticle was not discharged, a wet/dry vacuum was used to remove any residual particle on the water’s surface within each feeding ring, after 24 hours. Fish mortality was monitored daily for 96 hours, and floating fish were collected by hand, measured (fork length; millimeters), weighed (grams), and intestinal tracts were excised for yttrium tissue analysis. Intestinal tracts were frozen immediately for later processing at the U.S. Geological Survey Upper Midwest Environmental Sciences Center laboratory. Intestinal tracts were thawed in the laboratory and homogenized using dry ice and allowed to sublimate. Triplicate 0.5-g aliquots of homogenized individual fish intestines were acid digested according to EPA Method 3052 (U.S. Environmental Protection Agency, 1996) using a Mars 6 Microwave Digestion System (CEM Corporation, Matthews, North Carolina), and samples analytically verified for yttrium using an Agilent 5110 vertical dual view inductively coupled plasma optical emission spectrometer (Santa Clara, Calif.). After scanning a blank to account for sample background, standard solutions (1, 5, 10, 50, 100, 500, and 1,000 µg/L) and sample solutions were analyzed. The detection limit of yttrium (<1 µg/L) was determined by the concentration of yttrium, yielding a signal equivalent to three times the standard deviation of the blank signal. Instrument configuration and acquisition conditions are summarized in appendix 1. Triplicate intestinal samples per fish were used to calculate mean yttrium concentrations.
Results
The results of the study addressed the study objectives: (1) determined the acute toxicity of the antimycin microparticle formulation to invasive carp and non-target native fish when cohabitated in the laboratory, (2) determined the leaching rate of the antimycin microparticle in two water types, and (3) demonstrated that the microparticle delivery system establishes a toxicological selectivity for invasive carp across multiple experimental scales. All data associated with this study are available in Sauey and others (2024).
Laboratory Toxicity Studies
All fish survived in the experimental control test chambers. No differences or anomalies in water quality parameters were found and individual water quality parameters (dissolved oxygen, temperature, and pH) over the study duration were averaged (table 2). Going forward, the term “all fish” will refer to mortality observed in experimental chambers containing all four species per replicate. Individual species within each chamber will be referenced by common name to emphasize the differential toxicity observed among species. A dose-response curve was observed in each toxicity study with increasing mortality corresponding to an increasing type 2 antimycin concentration (milligrams per kilogram of total fish weight; fig. 2). Mortality was observed in all species at 6 hours, except largemouth bass. The antimycin microparticles caused an all fish mean (±SD) survival of 45.6 percent (±13.6) at the highest concentration (1.89 mg/kg) after 6 hours, and the lowest fish survival occurred in silver carp (10.0 percent), bighead carp (15.0 percent), and bluegill (42.5 percent; table 3; fig. 2). At 48 hours in the highest concentration, all fish survival was 23.1 percent (±5.5) and survival was 0 percent in silver carp and bighead carp, followed by 2.5 percent (±3.2), and 90.0 percent (±4.3) mortality in bluegill and largemouth bass, respectively (table 3). The all fish 6-, 24-, and 48-hour LD50 (confidence interval) values were 1.7 mg/kg (1.6–1.9), 1.0 mg/kg (0.8–1.3), and 0.9 mg/kg (0.7–1.2), respectively (table 4). Individual LD50 values were calculated for all species, except largemouth bass owing to inadequate fish mortality, and an inverse relationship between study duration and the derived LD50 value was observed, with derived LD50 values decreasing over time (table 4). Silver carp were the most sensitive to antimycin microparticle across all exposure durations (6–48 hours; fig. 3, table 4).
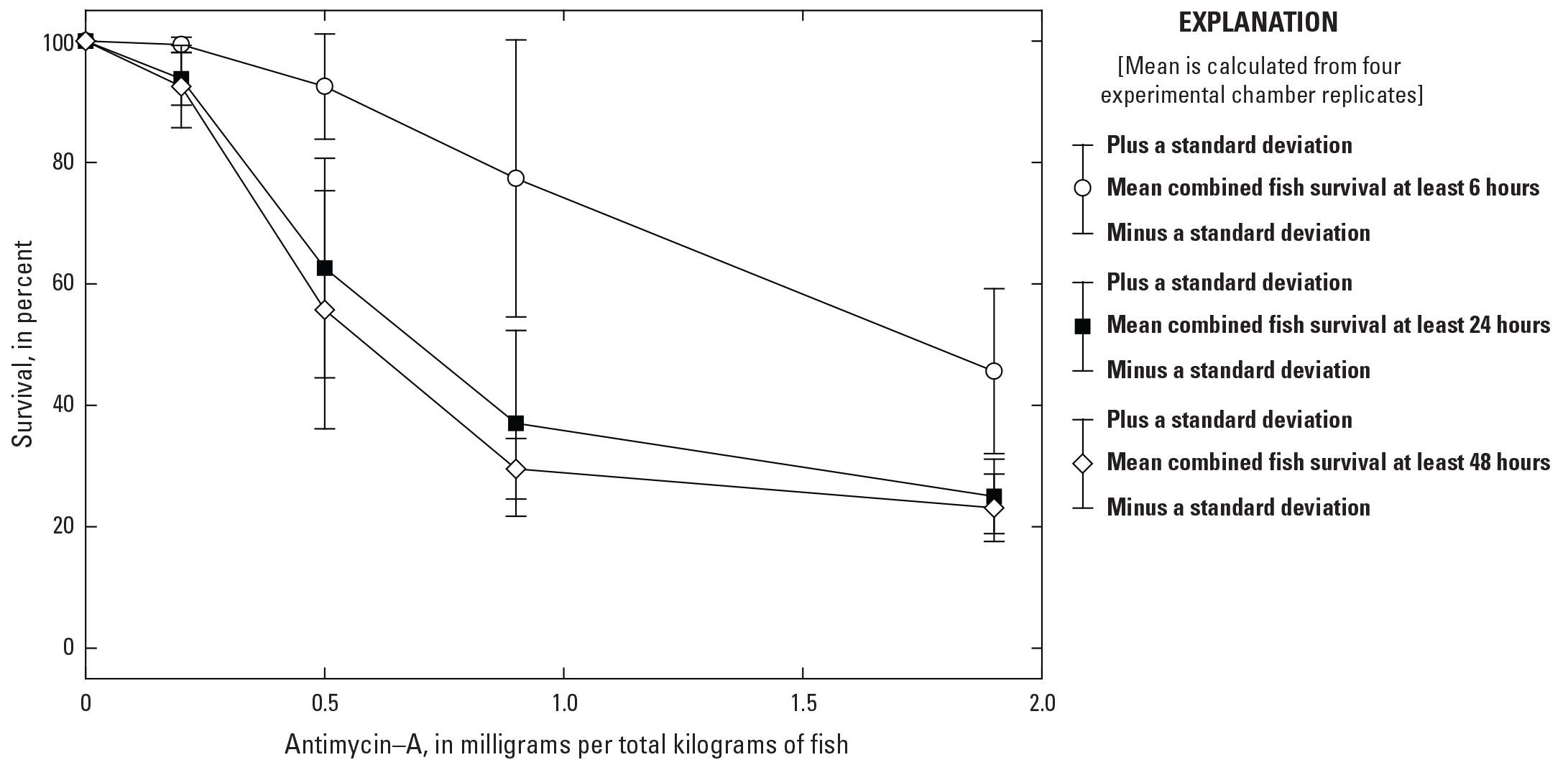
Mean combined fish survival with standard deviation at 6 hours, 24 hours, and 48 hours exposed to type 2 Antimycin–A microparticle (experimental chamber replicates=4). Experimental tanks contained four fish species (n=9–11 fish per species or 39–41 total fish): bighead carp (Hypophthalmichthys nobilis), silver carp (Hypophthalmichthys molitrix), bluegill (Lepomis macrochirus), and largemouth bass (Micropterus salmoides).

Mean fish survival with standard deviation for four fish species at 6 hours, 24 hours, and 48 hours exposed to type 2 Antimycin–A microparticle (experimental chamber replicates=4). Experimental tanks contained four fish species (n=9–11 fish per species): silver carp (Hypophthalmichthys molitrix), bighead carp (Hypophthalmichthys nobilis), bluegill (Lepomis macrochirus), and largemouth bass (Micropterus salmoides). The dose-response curves per species were plotted separately to visualize the temporal toxicity.
Table 4.
Laboratory-determined lethal dose values and 95-percent confidence intervals (parentheses) for fish exposed to type 2 Antimycin–A laden microparticle. Lethal dose values were calculated using the nominal concentrations (milligrams Antimycin–A per kilogram total fish weight). Four fish species (n=9–11) were present in each experimental chamber and the survival is represented by the term “All fish.” The survival of fish species within each experimental chamber is also represented by the species. Data summarized from Sauey and others (2024).[LD50, lethal dose to cause mortality in 50 percent of a population; CI, confidence interval; N/A, no value]
Laboratory Dissipation Study
The dissipation study demonstrated the presence and subsequent loss of antimycin in the water from the type 2 microparticle, but at significantly different rates per water type. The mean percent (±SD) dissipation after 24 hours in ultrapure water and Black River water was 52.9 percent (11.5) and 96.7 percent (1.2), respectively. Approximately 100 percent dissipation of antimycin was observed in ultrapure water after 33 days and in Black River water after 10 days. Linear regression analysis resulted in both water type’s slopes being significantly different than zero (ultrapure p-value <0.001, coefficient of determination (r2) = 0.85; Black River p-value <0.001, r2 = 0.92), and the dissipation rate (micrograms per liter per day; 95-percent confidence interval) of antimycin in ultrapure water was −0.2 (−0.1 to −0.2) and −0.4 (−0.4 to −0.5) in Black River water (table 5). The antimycin dissipation rate in Black River water was 2.6 times faster than in ultrapure water.
Table 5.
Type 2 Antimycin–A microparticle dissipation rate (micrograms per liter per day) and 95-percent confidence interval (parentheses) across two water types. Dissipation rate refers to the loss of antimycin in the water over time (days). Data summarized from Sauey and others (2024).[µg/L/day, microgram per liter per day; CI, confidence interval]
Experimental Pond Studies
The pond toxicity trials resulted in fish mortality following type 2 antimycin microparticle exposure at a larger experimental scale. In the first trial, no mortality was observed after 24 hours in the control (blank type 2 microparticle); however, significant mortality was observed in bighead carp (80 percent; p-value <0.001) and silver carp (25 percent; p-value=0.047) in the treated group exposed to type 2 microparticles. No largemouth bass (n=19) mortality was observed in the microparticle exposed group. In the second pond trial, no mortality was observed in the control group, and significant treated group mortality was observed in bighead carp (80 percent mortality; p-value <0.001), whereas insignificant mortality was observed in silver carp (20 percent mortality; p-value=0.106). Significant mortality was not observed in either the control or treatment groups for grass carp (n=10) and largemouth bass (n=10) 24 hours after application.
Rathbun Fish Hatchery Effluent Pond Field Study
Over the 2-week mark-recapture survey, only silver carp, river carpsucker, bigmouth buffalo, and smallmouth buffalo recaptures were sufficient to estimate abundance. Silver carp abundance (95-percent confidence interval) was estimated to be 1,593 (1,109–2,822), river carpsucker was 127 (80–301), smallmouth buffalo was 74 (44–242), and bigmouth buffalo was 9 (6–18). A total of 15 bighead carp and 6 common carp were captured, with only 1 recapture each. Captures of minimally sampled species were not reported.
The Rathbun Fish Hatchery effluent pond type 1 antimycin microparticle application resulted in fish mortality 96 hours after application, including 17 silver carp and 1 juvenile golden shiner (Notemigonus crysoleucas). The mean (±SD) silver carp mass was 3.6 (±0.6) kg. Inductively coupled plasma–optical emission spectrometer analysis of silver carp intestinal tracts indicated yttrium was present in all 17 dead silver carp. From the yttrium amounts measured in silver carp intestine samples, mean (±SD) microparticle consumed was 363.0 (±284.6) mg/kg of microparticle in fish and ranged from 98.2 to 1135.0 mg/kg of microparticle in fish. Based on the inclusion rate of yttrium and the nominal amount of antimycin used to generate the type 1 microparticle, the mean dose of antimycin delivered to the 17 silver carp was 73 mg/kg of antimycin in fish and ranged from 12 to 228 mg/kg of antimycin in fish. The total mean microparticle consumed by the 17 silver carp was estimated to be 18,304 mg or 3,680 mg antimycin (61 percent of the microparticle/antimycin added to the effluent pond).
Discussion
This study indicated different formulations of antimycin microparticles were lethal to filter-feeding silver and bighead carp across multiple experimental scales. The effectiveness and selectivity observed across studies with invasive and indigenous fish indicate that the microparticle application has the potential to be effective in targeting invasive carp in exposures containing fish with multiple feeding strategies; however, we did not test the life stages of non-target species that would consume a similar particle size. Whether omnivorous fish are susceptible to the antimycin microparticle following consumption needs to be carefully examined further to minimize the potential for nontarget fish lethality in prospective applications.
Microparticle technologies have been used in the aquaculture industry to deliver vitamins, minerals, and vaccines (Tobar and others, 2011; Caruffo and others, 2016). This study demonstrates that microparticle technology could be an effective mechanism for delivering a pesticide to filter-feeding fish and could be engineered to be selectively toxic to target fish species. Antimycin is a historical nonselective pesticide for nuisance fish removal and management (Gilderhus, 1972). Both antimycin microparticle formulations caused significant mortality to invasive carp compared to largemouth bass co-exposed in laboratory, and experimental pond studies. The antimycin microparticle oral LD50 values for fish are several magnitudes higher than those derived from water-based exposures, based on previous published acute Antimycin–A toxicity studies, which indicate encapsulated antimycin formulations decrease fish toxicity. The 24-hour laboratory antimycin microparticle LD50 value for bighead carp (0.74 mg/kg) is approximately 1,000 times higher than water-based 24-hour toxicity values (0.71–0.99 µg/L; Rach and others, 2009). Similarly, the bluegill 24-hour antimycin microparticle LD50 was 0.58 mg/kg compared to 0.13–0.92 µg/L during 24-hour water exposures (Mayer and Ellersieck, 1986). The selectivity associated with the microparticle technology was demonstrated by the lack of juvenile largemouth bass mortality observed across both laboratory and experimental pond studies because the water-based 24-hour antimycin LC50 values previously published for largemouth bass range from 0.37 to 1.02 µg/L (Mayer and Ellersieck, 1986). There is a margin of safety for indigenous fish when exposed to antimycin microparticles compared to antimycin water applications due to the characteristics of the microparticle formulation and the differences in fish feeding strategies, both of which were considered during microparticle formulation development.
As a proof-of-concept, the Rathbun Fish Hatchery study was successful at demonstrating that filter-feeding fish can be targeted using the antimycin microparticle. While only 1 percent of the silver carp population died from the pesticide application, the majority of the applied microparticles (61 percent) were consumed by only 17 fish. The development of application methods and delivery mechanisms that can disperse the microparticle to a greater proportion of the population will be necessary to increase effectiveness. With the exception of a single golden shiner (a species not previously detected), no mortality was observed in the non-target species known to be present. Further, non-laboratory research would be necessary to demonstrate selectivity with feral fish under conditions with similar fish abundances among target and non-target species, with an emphasis on native filter feeders (juvenile fish and invertebrates) associated with bighead and silver carp occurrence. However, given the invasive nature of these fish, a natural system suitable for testing antimycin microparticles would likely contain an unbalanced population structure, and silver carp would likely comprise the majority of the fish biomass (Sass and others, 2010) in locations where a targeted pesticide application would be a possible strategy.
Filter-feeding bighead carp diets vary based on zooplankton abundance and biomass (Kolar and others, 2005). Invasive carp are opportunistic consumers that can switch from zooplankton to phytoplankton based on seasonal biomass fluctuations, and a range of dietary food particle sizes have been reported ranging from 50 to 3,000 µm (Cremer and Smitherman, 1980; Spataru and others, 1983; Opuszynski and others 1991; Kolar and others, 2005). Wild juvenile bluegill (0 years old, 19–65 mm) diets mainly consist of small invertebrates (Dewey and others, 1997), which decreases the likelihood of antimycin microparticle consumption during a field application, although they are also opportunistic feeders. Largemouth bass were relatively unaffected by antimycin microparticle exposure, which may be attributed to the size and type of food they consumed at a very young age. Huskey and Turingan (2001) demonstrated largemouth bass adjust to piscivory at around 20–37 mm standard length, as soon as their mouth is big enough to consume larger prey and stop consuming plankton between 29 and 69 mm standard length. Furthermore, Wamboldt and others (2020) demonstrated that largemouth bass are top predators (that is, two trophic levels above plankton) at all size classes and essentially grow out of targeting plankton at a very young age. Therefore, the antimycin microparticle formulations evaluated in this study demonstrate that fish selectivity can be achieved using pesticide encapsulation technology to minimize indigenous fish mortality while causing significant invasive carp lethality at the laboratory, experimental pond, and effluent pond scales. Further development of the application method and microparticle dispersion can help increase the effectiveness of the microparticle as a pesticide delivery tool.
Owing to the acute toxicity of antimycin to fish following water-based exposures, the type 2 antimycin microparticle dissipation study was conducted to evaluate the occurrence of antimycin in water from the microparticle. Antimycin degrades via hydrolysis so developing an appropriate microparticle formulation that prevents immediate degradation is essential for direct application to water for consumption by a filter-feeding fish. However, due to its toxicity, the chemical cannot be allowed to leach from the microparticle and cause non-selective lethality indirectly before target fish consumption. The Black River water type 2 microparticle study demonstrated the mean dissipation (that is, loss) of the antimycin was 96.7 percent after 24 hours and confirms previous reports indicating antimycin rapidly degrades in the presence of water. Antimycin water dissipation was approximately 2 times slower in ultrapure water, indicating an influence of water constituents. Black River water is characterized as hard water (high hardness, alkalinity, and alkaline pH) and decreases the bioavailability of a weak acid such as antimycin (Saari, 2023). Lethality was observed following laboratory antimycin microparticle consumption by invasive carp and likely exposed fish to antimycin in the tank water. Minimal toxicity to largemouth bass was observed, which indicates antimycin water degradation is indeed quite rapid. Very few antimycin toxicity studies analytically verify exposure concentrations (Klimisch and others, 1997) and most report toxicity values based on nominal concentrations (Saari, 2023). The influence of pH on the degradation rate of antimycin has been demonstrated previously (Marking and Dawson, 1972); however, antimycin degradation was measured through fish bioassay and not through direct measurement of antimycin itself. Additional analytically verified dissipation studies across multiple water types, light and dark conditions, and temperatures are necessary to characterize antimycin dissipation rates from the microparticle in order to minimize the potential non-target fish mortality during microparticle applications. This study represents one of the first analytically verified dissipation rates for antimycin, which is in contrast to historical studies reporting nominal values and often monitoring degradation by means of biological activity (yeast or fish bioassay; Saari, 2023).
Microparticles that are similar have been shown to be effective tools at delivering vitamins and minerals to target species; however, the vitamins and minerals investigated are very water-soluble. Antimycin–A is very water-insoluble, and this may have caused a lower than predicted mortality when a wax microparticle was used. The digestive physiology (for example, enzymes) of carp may not have been sufficient to release enough antimycin from the wax particle to cause mortality, and only fish that consumed a large amount of microparticle were exposed to lethal levels owing to minimal partitioning of antimycin into water. Additional wax types in the microparticle formulation are planned to be examined. Reformulating the microparticle may have implications for aquaculture use but is beyond the scope of this report. For this technology to be useful for resource managers, additional field studies can be conducted to demonstrate outcome repeatability with indigenous fish, with an emphasis on filter-feeding fish, to comprehensively characterize species selectivity. Overall, the antimycin microparticle formulations evaluated in this study demonstrate that fish selectivity can be achieved using pesticide encapsulation technology to minimize indigenous fish mortality while causing invasive carp mortality at the laboratory, experimental pond, and effluent pond scales.
References Cited
Bernardy, J.A., Hubert, T.D., Ogorek, J.M., and Schmidt, L.J., 2013, Determination of Antimycin-A in water by liquid chromatographic/mass spectrometry—Single-laboratory validation: Journal of AOAC International, v. 96, no. 2, p. 413–421. [Also available at https://doi.org/10.5740/jaoacint.12-286.]
Caruffo, M., Maturana, C., Kambalapally, S., Larenas, J., and Tobar, J.A., 2016, Protective oral vaccination against infectious salmon anaemia virus in Salmo salar: Fish & Shellfish Immunology, v. 54, p. 54–59. [Also available at https://doi.org/10.1016/j.fsi.2016.03.009.]
Coulter, A.A., Keller, D., Amberg, J.J., Bailey, E.J., and Goforth, R.R., 2013, Phenotypic plasticity in the spawning traits of bigheaded carp (Hypophthalmichthys spp.) in novel ecosystems—Bigheaded carp phenotypic plasticity: Freshwater Biology, v. 58, no. 5, p. 1029–1037. [Also available at https://doi.org/10.1111/fwb.12106.]
Cremer, M.C., and Smitherman, R.O., 1980, Food habits and growth of silver and bighead carp in cages and ponds: Aquaculture, v. 20, no. 1, p. 57–64. [Also available at https://doi.org/10.1016/0044-8486(80)90061-7.]
Cudmore, B., Mandrak, N.E., Dettmers, J.M., Chapman, D.C., Kolar, C.S., 2012, Binational ecological risk assessment of bigheaded carps (Hypophthalmichthys spp.) for the Great Lakes Basin: Department of Fisheries and Oceans Canadian Science Advisory Secretariat, Research Document 2011/114, 13 p. [Also available at https://pubs.usgs.gov/publication/70039013.]
DeGrandchamp, K.L., Garvey, J.E., and Colombo, R.E., 2008, Movement and habitat selection by invasive Asian carps in a large river: Transactions of the American Fisheries Society, v. 137, no. 1, p. 45–56. [Also available at https://doi.org/10.1577/T06-116.1.]
Dewey, M.R., Richardson, W.B., and Zigler, S.J., 1997, Patterns of foraging and distribution of bluegill sunfish in a Mississippi River backwater—Influence of macrophytes and predation: Ecology Freshwater Fish, v. 6, no. 1, p. 8–15. [Also available at https://doi.org/10.1111/j.1600-0633.1997.tb00137.x.]
Finlayson, B.J., Schnick, R.A., Cailteux, R.L., DeMong, L., Horton, W.D., McClay, W., and Thompson, C.W., 2002, Assessment of Antimycin a use in fisheries and its potential for reregistration: Fisheries, v. 27, no. 6, p. 10–18. [Also available at https://doi.org/10.1577/1548-8446(2002)027%3C0010:AOAAUI%3E2.0.CO;2.]
Fredricks, K.T., Hubert, T.D., Amberg, J.J., Cupp, A.R., and Dawson, V.K., 2021, Chemical controls for an integrated pest management program: North American Journal of Fisheries Management, v. 41, no. 2, p. 289–300. [Also available at https://doi.org/10.1002/nafm.10339.]
Gaikowski, M.P., 2018, Asian carp control: U.S. Department of the Interior web page, accessed August 1, 2018, at https://www.doi.gov/ocl/asian-carp-control.
Gilderhus, P.A., 1972, Exposure times necessary for antimycin and rotenone to eliminate certain freshwater fish: Journal of the Fisheries Research Board of Canada, v. 29, no. 2, p. 199–202. [Also available at https://doi.org/10.1139/f72-032.]
Hawkyard, M., Laurel, B., and Langdon, C., 2014, Rotifers enriched with taurine by microparticulate and dissolved enrichment methods influence the growth and metamorphic development of northern rock sole (Lepidopsetta polyxystra) larvae: Aquaculture, v. 424–425, p. 151–157. [Also available at https://doi.org/10.1016/j.aquaculture.2013.12.035.]
Hawkyard, M., Sæle, Ø., Nordgreen, A., Langdon, C., and Hamre, K., 2011, Effect of iodine enrichment of Artemia sp. on their nutritional value for larval zebrafish (Danio rerio): Aquaculture, v. 316, no. 1–4, p. 37–43. [Also available at https://doi.org/10.1016/j.aquaculture.2011.03.013.]
Huskey, S.H., and Turingan, R.G., 2001, Variation in prey-resource utilization and oral jaw gape between two populations of largemouth bass, Micropterus salmoides: Environmental Biology of Fishes, v. 61, no. 2, p. 185–194. [Also available at https://doi.org/10.1023/A:1011095526939.]
Jobling, M., Covés, D., Damsgård, B., Kristiansen, H.R., Koskela, J., Petursdottir, T.E., Kadri, S., and Gudmundsson, O., 2001, Techniques for measuring feed intake, chap. 3 of Houlihan, D., Boujard, T., and Jobling, M., Food intake in fish: New York, Wiley, p. 49–87, accessed November 2023, at https://doi.org/10.1002/9780470999516.ch3.
Klimisch, H.-J., Andreae, M., and Tillmann, U., 1997, A systematic approach for evaluating the quality of experimental toxicological and ecotoxicological data: Regulatory Toxicology and Pharmacology, v. 25, no. 1, p. 1–5. [Also available at https://doi.org/10.1006/rtph.1996.1076.]
Kolar, C.S., and Morrison, S.S., 2016, USGS science and technology help managers battle invading Asian carp: U.S. Geological Survey Fact Sheet 2016–3063, 4 p. [Also available at https://doi.org/10.3133/fs20163063.]
Langdon, C., Nordgreen, A., Hawkyard, M., and Hamre, K., 2008, Evaluation of wax spray beads for delivery of low-molecular weight, water-soluble nutrients and antibiotics to Artemia: Aquaculture, v. 284, no. 1–4, p. 151–158. [Also available at https://doi.org/10.1016/j.aquaculture.2008.07.032.]
Mallison, C.T., Hestand, R.S., III, and Thompson, B.Z., 1995, Removal of triploid grass carp with an oral Rotenone bait in two Central Florida lakes: Lake and Reservoir Management, v. 11, no. 4, p. 337–342. [Also available at https://doi.org/10.1080/07438149509354215.]
Marking, L.L., and Dawson, V.K., 1972, The half-life of biological activity of Antimycin determined by fish bioassay: Transactions of the American Fisheries Society, v. 101, no. 1, p. 100–105, accessed January 2023 at https://doi.org/10.1577/1548-8659(1972)101<100:THOBAO>2.0.CO;2.
Opuszynski, K., Shireman, J.V., and Cichra, C.E., 1991, Food assimilation and filtering rate of bighead kept in cages: Hydrobiologia, v. 220, no. 1, p. 49–56. [Also available at https://doi.org/10.1007/BF00017491.]
Pendleton, R.M., Schwinghamer, C., Solomon, L.E., and Casper, A.F., 2017, Competition among river planktivores—Are native planktivores still fewer and skinnier in response to the Silver Carp invasion?: Environmental Biology of Fishes, v. 100, no. 10, p. 1213–1222, accessed November 2023 at https://doi.org/10.1007/s10641-017-0637-7.
Poole, J.R., Sauey, B.W., Amberg, J.J., and Bajer, P.G., 2018, Assessing the efficacy of corn-based bait containing antimycin-a to control common carp populations using laboratory and pond experiments: Biological Invasions, v. 20, no. 7, p. 1809–1820. [Also available at https://doi.org/10.1007/s10530-018-1662-y.]
Rach, J.J., Luoma, J.A., and Marking, L.L., 1994, Development of an Antimycin‐impregnated bait for controlling common carp: North American Journal of Fisheries Management, v. 14, no. 2, p. 442–446. [Also available at https://doi.org/10.1577/1548-8675(1994)014%3C0442:DOAAIB%3E2.3.CO;2.]
Rach, J.J., Boogaard, M., and Kolar, C., 2009, Toxicity of rotenone and antimycin to silver carp and bighead carp: North American Journal of Fisheries Management, v. 29, no. 2, p. 388–395. [Also available at https://doi.org/10.1577/M08-081.1.]
Saari, G.N., 2023, Antimycin-A species sensitivity distribution—Perspectives for non-indigenous fish control: Management of Biological Invasions, v. 14, no. 3, p. 503–517. [Also available at https://doi.org/10.3391/mbi.2023.14.3.09.]
Sampson, S.J., Chick, J.H., and Pegg, M.A., 2009, Diet overlap among two Asian carp and three native fishes in backwater lakes on the Illinois and Mississippi rivers: Biological Invasions, v. 11, no. 3, p. 483–496. [Also available at https://doi.org/10.1007/s10530-008-9265-7.]
Sass, G.G., Cook, T.R., Irons, K.S., McClelland, M.A., Michaels, N.N., Matthew O’Hara, T., and Stroub, M.R., 2010, A mark-recapture population estimate for invasive silver carp (Hypophthalmichthys molitrix) in the La Grange Reach, Illinois River: Biological Invasions, v. 12, no. 3, p. 433–436. [Also available at https://doi.org/10.1007/s10530-009-9462-z.]
Sass, G.G., Hinz, C., Erickson, A.C., McClelland, N.N., McClelland, M.A., and Epifanio, J.M., 2014, Invasive bighead and silver carp effects on zooplankton communities in the Illinois River, Illinois, USA: Journal of Great Lakes Research, v. 40, no. 4, p. 911–921. [Also available at https://doi.org/10.1016/j.jglr.2014.08.010.]
Sauey, B.W., Saari, G.N., Putnam, J.G., Nelson, J.E., Wamboldt, J.J., Steiner, J.N., and Calfee, R.D., 2024, Data release for a novel tool to selectively deliver a control agent to filter-feeding silver and bighead carp: U.S. Geological Survey data release, https://doi.org/10.5066/P1DHXFTG.
Schrank, S.J., and Guy, C.S., 2002, Age, growth, and gonadal characteristics of adult bighead carp, Hypophthalmichthys nobilis, in the Lower Missouri River: Environmental Biology of Fishes, v. 64, no. 4, p. 443–450. [Also available at https://doi.org/10.1023/A:1016144529734.]
Spataru, P., Wohlfarth, G.W., and Hulata, G., 1983, Studies on the natural food of different fish species in intensively manured polyculture ponds: Aquaculture, v. 35, p. 283–298. [Also available at https://doi.org/10.1016/0044-8486(83)90101-1.]
Taverna, M.E., Busatto, C.A., Lescano, M.R., Nicolau, V.V., Zalazar, C.S., Meira, G.R., and Estenoz, D.A., 2018, Microparticles based on ionic and organosolv lignins for the controlled release of atrazine: Journal of Hazardous Materials, v. 359, p. 139–147. [Also available at https://doi.org/10.1016/j.jhazmat.2018.07.010.]
Tobar, J.A., Jerez, S., Caruffo, M., Bravo, C., Contreras, F., Bucarey, S.A., and Harel, M., 2011, Oral vaccination of Atlantic salmon (Salmo salar) against salmonid rickettsial septicaemia: Vaccine, v. 29, no. 12, p. 2336–2340. [Also available at https://doi.org/10.1016/j.vaccine.2010.12.107.]
U.S. Army Corps of Engineers and others, 2023, Upper Mississippi River Restoration Program—Long Term Resource Monitoring: accessed December 2023 at https://umesc.usgs.gov/ltrm-home.html.
Walleser, L.R., Sandheinrich, M.B., Howard, D.R., Gaikowski, M.P., and Amberg, J.J., 2014, Spatial and temporal variation of the gill rakers of gizzard shad and silver carp in three midwestern rivers: North American Journal of Fisheries Management, v. 34, no. 5, p. 875–884. [Also available at https://doi.org/10.1080/02755947.2014.920740.]
Wamboldt, J.J., Wanamaker, A.D., Jr., Carroll, H.M., Schultz, R.D., and Morris, J.E., 2020, Trophic dynamics of a reservoir fishery following an introduction of a top predator—Insights from stable carbon and nitrogen isotopes: Fisheries Management and Ecology, v. 27, no. 5, p. 531–539. [Also available at https://doi.org/10.1111/fme.12445.]
Wang, W., Ge, J., and Yu, X., 2020, Bioavailability and toxicity of microplastics to fish species—A review: Ecotoxicology and Environmental Safety, v. 189, article 109913, 10 p. [Also available at https://doi.org/10.1016/j.ecoenv.2019.109913.]
Appendix 1
Liquid Chromatography-Quadrupole Time of Flight Method
An Agilent Technologies (Santa Clara, California, United States) Liquid Chromatography-Quadrupole Time of Flight system was used to measure and analyze the concentration of Antimycin–A in water samples. This system consisted of an Agilent 1290 Infinity Liquid Chromatography stack (G1330B mobile phase pump, G4226A autosampler, G1316C column heater, and G1314E UV detector) and Agilent 6530 Accuate-Mass Quadrupole Time of Flight. The separation of the four homologs was achieved on an Agilent Zorbax Extended C18 Rapid Resolution 2.1x100 millimeters 1.8 micrometers column. The mobile phase solutions consisted of Mobile Phase A (5 millimolar [mM] ammonium acetate at pH 8.5 in 50:50 ratio of ultrapure water:methanol) and Mobile Phase B (methanol). The mobile phase gradient used is shown in table 1.1.
Table 1.1.
A time table describing the change in liquid chromatography mobile phases (A and B) throughout the acquisition. The mobile phases consisted of Mobile Phase A (5 millimolar [mM] ammonium acetate at pH 8.5 in 50:50 ratio of ultrapure water:methanol) and Mobile Phase B (methanol).The mobile phase flow rate was 0.25 milliliter per minute with an analysis run time of 6.0 minutes. The column temperature was kept at a constant 35 degrees Celsius (°C). The injection volume was 1 microliter (µL) for samples and 10 µL for the standards solutions. The Quadrupole Time of Flight Mass Spectrometer conditions are shown in table 1.2, and the ion selection and detection parameters are shown in table 1.3. All chromatographic data were processed with Agilent’s MassHunter software to determine sample concentration.
Table 1.2.
The Quadrupole Time of Flight Mass Spectrometer conditions for the Antimycin–A quantitation.Liquid Chromatography-Triple Quadrupole Mass Spectrometer Method
An Agilent Technologies (Santa Clara, California, United States) Liquid Chromatography-Triple Quadrupole Mass Spectrometer system was used to measure and analyze the Antimycin–A concentration in water samples. This system consisted of an Agilent 1260 Infinity Liquid Chromatography stack (G1379B mobile phase degasser, G1312B mobile phase pump, G1329B autosampler, G1316B column heater, and G4212B UV detector) and Agilent 6460 Triple Quadrupole Mass Spectrometer. Antimycin–A homolog separation was achieved on an Agilent Zorbax Eclipse Plus C18 2.1x100 millimeters 1.8 micrometer column. The mobile phase solutions consisted of Mobile Phase A (5 mM ammonium acetate at pH 8.5 in 50:50 ratio of ultrapure water:methanol) and Mobile Phase B (methanol). The mobile phase gradient used throughout the acquisition is shown in table 1.4.
Table 1.4.
A timetable describing the change in liquid chromatography mobile phases (A and B) throughout the acquisition. The mobile phases consisted of Mobile Phase A (5 millimolar [mM] ammonium acetate at pH 8.5 in 50:50 ratio of ultrapure water:methanol) and Mobile Phase B (methanol).The mobile phase flow rate was 0.3 milliliter per minute with an analysis run time of 3.5 minutes, and the column temperature was kept constant at 35 °C. The injection volume was 1 µL for samples and 10 µL for the standards solutions. The Triple Quadrupole Mass Spectrometer conditions are shown in table 1.5.
The ion selection and detection parameters are shown in table 1.6. The chromatographic data was processed with Agilent’s MassHunter software to determine sample concentrations.
Inductively Coupled Plasma–Optical Emission Spectrometer Method
An Agilent 5110 VDV inductively coupled plasma–optical emission spectrometer instrument was used for yttrium analysis. A 1,000-microgram per liter (µg/L) Scandium reference solution was used as an internal standard during analysis (361.383 nanometers). After scanning a blank to account for sample background, standard solutions (1, 5, 10, 50, 100, 500, and 1,000 µg/L) and all sample solutions were analyzed. The instrument configuration and experimental conditions are summarized in table 1.7. For each sample, triplicate determinations were performed and mean results were reported. The detection limit of yttrium (less than 1 µg/L) in samples was determined from the standard curve containing yttrium in the samples with 5-percent nitric acid in water based on the usual definition as the concentration of the analyte yielding a signal equivalent to three times the standard deviation of the blank signal. The detection limits of the method are sufficient and permit the determination of yttrium in 5-percent nitric acid in water at background concentrations.
Table 1.7.
Inductively coupled plasma–optical emission spectrometer operating conditions.Conversion Factors
International System of Units to U.S. customary units
Temperature in degrees Celsius (°C) may be converted to degrees Fahrenheit (°F) as follows:
°F = (1.8 × °C) + 32.
Supplemental Information
Concentrations of chemical constituents in water are in either milligrams per liter (mg/L) or micrograms per liter (µg/L). Concentrations of chemical constituents in fish are given in milligrams per kilogram (mg/kg).
For more information about this publication, contact:
Director, Upper Midwest Environmental Sciences Center
U.S. Geological Survey
2630 Fanta Reed Road
La Crosse, WI 54603
For additional information, visit: https://www.usgs.gov/centers/upper-midwest-environmental-sciences-center
Publishing support provided by the Rolla Publishing Service Center
Disclaimers
Any use of trade, firm, or product names is for descriptive purposes only and does not imply endorsement by the U.S. Government.
Although this information product, for the most part, is in the public domain, it also may contain copyrighted materials as noted in the text. Permission to reproduce copyrighted items must be secured from the copyright owner.
Suggested Citation
Sauey, B.W., Saari, G.N., Putnam, J.G., Nelson, J.E., Wamboldt, J.J., Steiner, J.N., and Calfee, R.D., 2024, A novel tool to selectively deliver a control agent to filter-feeding silver and bighead carp: U.S. Geological Survey Open-File Report 2024–1052, 17 p., https://doi.org/10.3133/ofr20241052.
ISSN: 2331-1258 (online)
Publication type | Report |
---|---|
Publication Subtype | USGS Numbered Series |
Title | A novel tool to selectively deliver a control agent to filter-feeding silver and bighead carp |
Series title | Open-File Report |
Series number | 2024-1052 |
DOI | 10.3133/ofr20241052 |
Year Published | 2024 |
Language | English |
Publisher | U.S. Geological Survey |
Publisher location | Reston, VA |
Contributing office(s) | Columbia Environmental Research Center, Upper Midwest Environmental Sciences Center |
Description | Report: vii, 17 p.; Data Release |
Online Only (Y/N) | Y |
Additional Online Files (Y/N) | N |
Google Analytic Metrics | Metrics page |