Depth to Water and Water Quality in Groundwater Wells in the Ogallala Aquifer Within the North Plains Groundwater Conservation District, Texas Panhandle, 2019–20, and Comparison to 2012–13 Conditions
Links
- Document: Report (6.79 MB pdf) , HTML , XML
- Dataset: USGS National Water Information System database —USGS water data for the Nation
- Data Release: USGS data release - Water-quality and depth to water for groundwater wells primarily completed in the Ogallala aquifer within the North Plains Groundwater Conservation District, Texas Panhandle, 2012–13 and 2019–20
- Download citation as: RIS | Dublin Core
Acknowledgments
The authors thank North Plains Groundwater Conservation District employees Louis Orthman and Odell Ward for their assistance with data collection efforts and for coordinating access to sites throughout the sampling period.
Abstract
The Ogallala aquifer is the primary source of water for agricultural and municipal purposes in the Texas Panhandle. Because most of the groundwater in the Texas Panhandle is withdrawn from the Ogallala aquifer, information on the quality of groundwater in the Ogallala aquifer in this part of Texas is useful for resource characterization. During 2012–13, the U.S. Geological Survey in cooperation with the North Plains Groundwater Conservation District (NPGCD), collected and analyzed water-quality samples from 30 groundwater monitoring wells in the Texas Panhandle. The results of the initial 2012–13 synoptic sampling were published in 2014 to help provide an initial characterization of the spatial and temporal variability of water quality in the NPGCD management area. This report documents the results of a followup synoptic sampling completed between March 2019 and July 2020 by the U.S. Geological Survey, in cooperation with the NPGCD, to further characterize the spatial and temporal characteristics of groundwater in the NPGCD management area; measurements of the depth to water, in feet below land surface, and water-quality samples were obtained from the same 30 monitoring wells that were sampled during 2012–13. The water-quality samples were analyzed for major ions, nutrients, trace elements, and selected organic compounds. Results from the 2019–20 synoptic sampling were compared to drinking-water standards and to the results from the 2012–13 synoptic sampling.
Between the 2012–13 and 2019–20 sampling periods, the depth to water increased in 28 of the 30 wells, with a median difference of 18.17 feet. Results from major ion analyses indicate that most of the groundwater samples collected during 2019–20 were classified as magnesium-bicarbonate type, the same water type indicated for most samples during 2012–13. Dissolved-solids concentrations for the wells sampled during 2019–20 ranged from 260 to 774 milligrams per liter (mg/L) with a median dissolved-solids concentration of 316 mg/L, which was slightly higher than the median dissolved-solids concentration of 311 mg/L for the 2012–13 sampling period. Of the four nutrients analyzed, nitrate was the dominant nitrogen species, with a median nitrate concentration of 2.25 mg/L for the 2019–20 sampling period, which was a slight increase relative to the median nitrate concentration of 2.05 mg/L for the 2012–13 sampling period. Accounting for variability in analyses, median major ion concentrations and median concentrations for nutrient species were similar during the 2012–13 and 2019–20 sampling periods. None of the trace element concentrations exceeded any maximum contaminant level or secondary drinking-water standards. Median concentrations of trace elements from the 2012–13 sampling period were compared to those from the 2019–20 sampling period for constituents in cases where at least 50 percent of concentrations measured in the samples were detected at concentrations greater than the highest applicable laboratory reporting level, and variability in analyses was accounted for. Comparison results indicated that that median concentrations of two trace elements (lithium and uranium) increased, whereas median concentrations for two of the other trace elements measured (barium and molybdenum) decreased. Atrazine and deethylatrazine were the only organic compounds detected; both were detected in four of the six samples collected from different wells and analyzed for organic compounds. Concentrations of atrazine and deethylatrazine detections were all less than 0.05 micrograms per liter.
Introduction
The Ogallala aquifer is part of the High Plains aquifer system, which is an extensively used groundwater system underlying parts of Colorado, Kansas, Nebraska, New Mexico, Oklahoma, South Dakota, Wyoming, and Texas (fig. 1; McGuire, 2017). The High Plains aquifer system is commonly divided into three parts (northern, central, and southern). The northern High Plains aquifer system underlies most of Nebraska and parts of Colorado, Kansas, South Dakota, and Wyoming. The central High Plains aquifer system underlies a large part of Kansas and parts of Colorado, New Mexico, Oklahoma, and the Texas Panhandle. The southern High Plains aquifer system underlies northern Texas, mainly south of the Canadian River, and extends into eastern New Mexico (Becker and others, 2002).
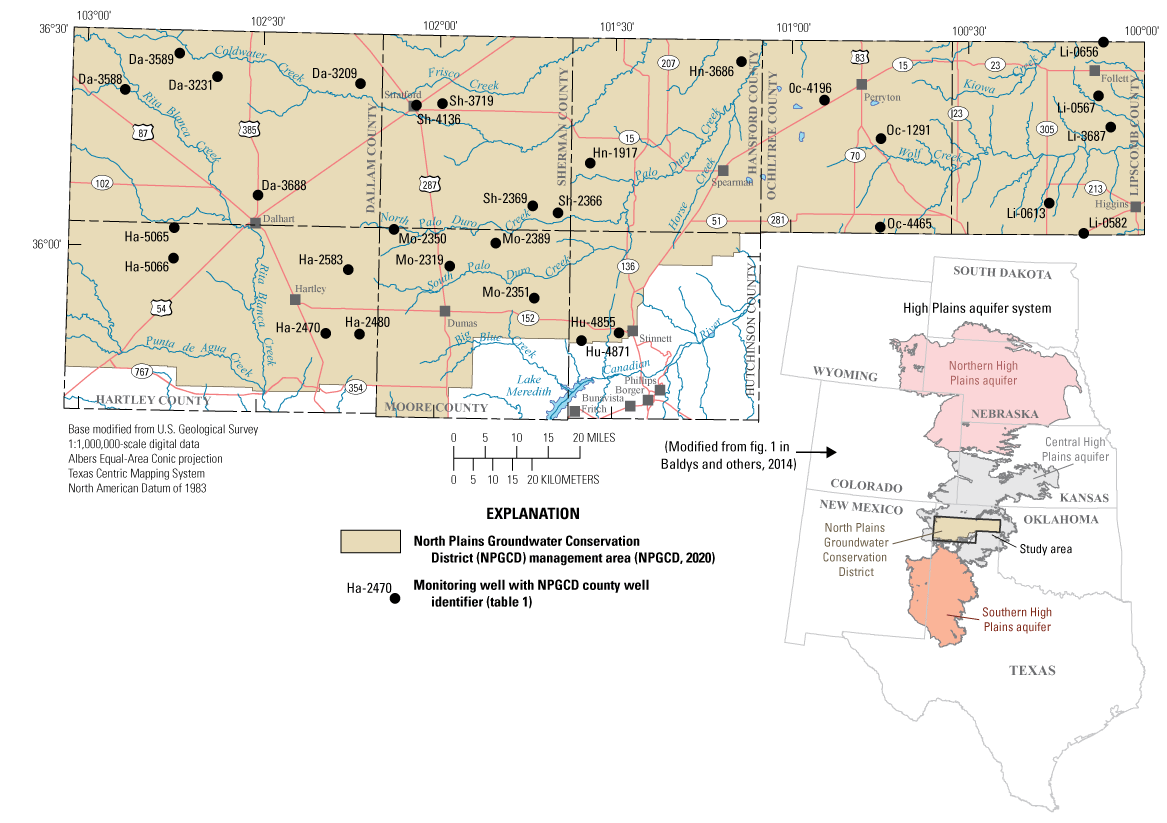
Study area and groundwater sampling locations in the Ogallala aquifer (part of the High Plains aquifer system) in the Texas Panhandle for the 2019–20 synoptic sampling.
The study area for this report coincides with the North Plains Conservation District (NPGCD) management area in the northern part of the Texas Panhandle (fig. 1). In the study area, groundwater withdrawn from the central High Plains aquifer system is extracted from the Ogallala aquifer. Communities in the study area rely on the Ogallala aquifer as their primary source of water for agricultural and municipal purposes. Because most of the groundwater in the study area is withdrawn from the Ogallala aquifer, information on the quality of groundwater in the Ogallala aquifer is useful for resource characterization. Information on the concentrations of inorganic and organic compounds in water samples obtained from the Ogallala aquifer can provide insights on the suitability of the groundwater for various uses and for making other water-management decisions. General groundwater-quality characteristics, such as the concentrations of dissolved solids, nutrients, and trace elements, help in understanding how the quality of groundwater in the Ogallala aquifer varies spatially and temporally. Knowledge of the concentrations of organic compounds, such as pesticides and herbicides and their degradates, in the Ogallala aquifer also is important for groundwater characterization and management purposes.
The NPGCD, created by petition to the State Board of Water Engineers in 1954 (NPGCD, 2020), has regulatory authority for the central High Plains aquifer in parts the Texas Panhandle north of the Canadian River (fig. 1). The NPGCD management area covers 7,390 square miles and is charged by the Texas Water Development Board with managing groundwater in the Ogallala aquifer for the conservation, protection, and preservation of this valuable resource as stated in their mission statement, “maintaining our way of life through conservation, protection, and preservation of our groundwater resources” (NPGCD, 2020, p. 5).
During 2012–13, the U.S. Geological Survey (USGS) in cooperation with the NPGCD, collected and analyzed water-quality samples from 30 groundwater monitoring wells in the NPGCD in the Texas Panhandle. The results of this initial synoptic sampling study were previously published (Baldys and others, 2014) to help characterize the spatial and temporal variability of water quality in the NPGCD management area. A need for additional data was recognized by the NPGCD; the limited available data on the quality of water in the Ogallala aquifer make monitoring temporal variability and determining the availability and suitability of this vital resource for various uses difficult (NPGCD, 2020). Therefore, the USGS, in cooperation with the NPGCD, completed another synoptic sampling to better characterize groundwater quality in the Ogallala aquifer in northern part of the Texas Panhandle. The second synoptic sampling was completed between March 2019 and July 2020 to provide insights into the spatial and temporal characteristics of groundwater in the NPGCD management area. Depth to water-level measurements were made and water-quality samples were collected and analyzed for major ions, nutrients, trace elements, and selected organic compounds from the same set of 30 monitoring wells that were sampled during 2012–13 (Baldys and others, 2014). All of the wells used in the study were designated monitoring wells within the NPGCD management area and are part of a groundwater monitoring network maintained by the NPGCD. The term “designated monitoring well” refers to a well that is used exclusively for monitoring groundwater levels and collecting groundwater-quality samples to characterize groundwater resources.
Purpose and Scope
The purpose of this report is to document the results of a synoptic sampling done by the USGS in cooperation with the NPGCD during 2019–20 and compare the results to those of a previous synoptic sampling conducted during 2012–13 (Baldys and others, 2014). Depth to water-level measurements and groundwater-quality samples were collected from 30 monitoring wells completed in the Ogallala aquifer in the NPGCD management area as part of this synoptic sampling (fig. 1; table 1). Groundwater-quality samples were collected for the analysis of dissolved solids, major ions, nutrients, and trace elements; selected organic compounds also were analyzed in 6 of the 30 wells. Results from the 2019–20 sampling period for selected constituents were compared to U.S. Environmental Protection Agency (EPA) drinking-water standards and to the 2012–13 sampling results. The groundwater-quality data from the samples collected were also assessed in the context of results obtained from quality-control samples collected in conjunction with the environmental samples.
Table 1.
Wells completed in the Ogallala aquifer in the Texas Panhandle that were sampled during 2019–20.[USGS, U.S. Geological Survey; NPGCD, North Plains Groundwater Conservation District]
Description of Study Area
The study area for this report coincides with the area managed by the NPGCD, which consists of the five northernmost counties that form the border between Texas and Oklahoma (Dallam, Sherman, Hansford, Ochiltree, and Lipscomb Counties) and parts of Hartley, Moore, and Hutchinson Counties north of the Canadian River where the rocks that contain the Ogallala aquifer are present at land surface. (fig. 1).
During 1981–2010, the average annual precipitation for the 7,390-square-mile study area ranged from 15.7 inches (in.) at Dalhart, Texas (Dallam County), in the western part of the study area to 23.2 in. in Follett, Tex. (Lipscomb County), in the eastern part of the study area (National Oceanic and Atmospheric Administration, 2021). In the study area, the NPGCD estimates that average annual recharge rates are less than one-third of an inch per year (NPGCD, 2020). Most of this negligible amount of recharge is from precipitation infiltrating through streambeds and playas and eventually reaching the groundwater table (Scanlon and others, 2002; McGuire, 2017).
Geologic and Hydrogeologic Setting
In the study area, the Ogallala aquifer is contained in the Ogallala Formation, a sand and gravel deposit capped with caliche. The Ogallala Formation was deposited during the Miocene Epoch of the Tertiary Period (Gutentag and others, 1984; Ryder, 1996). The Ogallala Formation is the principal water-bearing geologic unit of the central High Plains aquifer (Gutentag and others, 1984; Ryder, 1996); historically, the High Plains aquifer system was often referred to as the “Ogallala aquifer” throughout its extent in reference to the predominant water-bearing unit of the aquifer system, the Ogallala Formation (Dugan and others, 1994). In places, the Ogallala aquifer is in hydraulic connection with permeable parts of the underlying bedrock, which ranges in age from Permian to Cretaceous (Ryder, 1996). Regional uplift and erosion have stripped away the Great Plains (Ogallala Formation) in many places, but a large central area was little affected by eroding streams and is preserved. This preserved remnant of the uplifted area that contains the Ogallala Formation is known as the High Plains. Although the land surface of the High Plains has been modified little by streams, it has been pitted by carbonate dissolution and deflation, thus forming many playas (shallow depressions) that collect and store water during periods of precipitation and runoff. (Ryder, 1996). The Canadian River has cut through much of the Ogallala Formation in the Texas Panhandle. The Ogallala Formation consists primarily of heterogeneous sequences of coarse-grained sand and gravel, which originated from alluvial fan deposits and fluvial, lacustrine, and eolian deposits (NPGCD, 2020; Ryder, 1996). The NPGCD reports that the average thickness of the Ogallala Formation in the study area ranges from 115 ft in Moore County to 225 ft in Lipscomb County, with an estimated study-area-wide average aquifer thickness of 148 ft (NPGCD, 2020).
Data Collection and Analysis Methods
After measuring the depth to water at each of the 30 monitoring wells, selected physicochemical properties were measured at the same time groundwater-quality samples were collected and processed as part of the synoptic study. The physicochemical properties measured onsite were dissolved oxygen, pH, specific conductance, groundwater temperature, turbidity, and alkalinity. Water-quality samples were analyzed for dissolved solids, nutrients, trace elements, and organic compounds. All water-quality results including physicochemical properties were reviewed for completeness and accuracy and are stored in the National Water Information System (USGS, 2021) and are also published in a companion data release (Ging and Mobley, 2021).
Depth to Water Level Methods
The same methods used in the previous synoptic study (Baldys and others, 2014) by USGS personnel to make depth to water-level measurements and collect water-quality samples were used in this study. At each of the monitoring wells shown in figure 1, the depth to water was measured before collecting water-quality samples to help ensure the sampling hose intake was at the proper depth to provide a representative sample and to determine any changes in groundwater levels (fig. 1; Ging and Mobley, 2021). The depth to water was measured at most wells by using USGS-owned electric groundwater-level measurement tapes (electric tapes or e-tapes) in accordance with methods described in Cunningham and Schalk (2011). The electric tapes used by USGS personnel were calibrated before use with a steel reference tape. An electric tape commonly is accurate to plus or minus 0.01 ft and is most accurate for groundwater levels less than 200 ft below land surface (Cunningham and Schalk, 2011). Depths to water in two of the wells where the groundwater depth exceeded 500 ft were measured by USGS personnel with a NPGCD-owned electric tape that could measure groundwater depths as great as 750 ft below land surface; the electric tape belonging to NPGCD was not subjected to the same reference calibration checks as the USGS-owned electric tape that was used.
NPGCD county well identifiers assigned to each well were used to identify the wells in this report and typically consist of the first two letters of the county name followed by a sequence of four numbers ( Baldys and others, 2014; NPGCD, 2020; Ging and Mobley, 2021). The county well identifiers assigned by the NPGCD supplement the 15-digit USGS station numbers used to identify each well in the National Water Information System database (table 1; USGS, 2021).
Water-Quality Sample Collection and Processing
Water-quality samples were collected from each of the 30 wells and processed onsite. Quality-control samples consisting of blank samples, replicate samples, and spike samples were collected to assess bias and precision in sampling and processing procedures. Groundwater-quality samples were processed in a mobile water-quality laboratory by following procedures outlined in the USGS “National Field Manual for the Collection of Water-Quality Data” (USGS, variously dated). A submersible pump was lowered into each well and was attached to stainless steel pipe sections to pump the groundwater directly from the wells to a manifold, which was used to direct the water to polytetrafluoroethylene (PTFE) tubing line that was routed into the mobile water-quality laboratory for sample processing.
Each well was purged to ensure that samples collected for analyses were representative of the groundwater in the aquifer. During the purging process, selected physicochemical properties (dissolved oxygen, pH, specific conductance, groundwater temperature, and turbidity) were measured and recorded as the unfiltered groundwater was pumped from the well. Standard USGS procedures require monitoring these physicochemical properties by using a multisensor meter installed in an airtight flow chamber until readings for the physical properties stabilize (USGS, variously dated). Each well was purged of at least three well casing volumes of groundwater prior to sample collection.
Once the physicochemical properties had stabilized, the well discharge was redirected to an enclosed processing chamber in the mobile water-quality laboratory where groundwater samples were processed for analysis. Sample collection and preservation were completed in the processing chamber to prevent the introduction of airborne contaminants. The tubing and fittings used for sample collection and processing were made of stainless steel or PTFE. The bottles used for sample collection were made of baked glass or polypropylene. The bottles were rinsed with deionized water prior to sample collection. Sampling equipment was thoroughly cleaned after each well was sampled following standard USGS procedures (USGS, variously dated). The stainless-steel pipe sections used to lower the submersible pump into the well were washed between sites with a pressurized water spray and then rinsed with deionized water (a high-volume rinse). Pipe sections were capped at each end during transport to prevent the introduction of dust and other airborne contaminants. Samples analyzed for major ions, trace elements, and nutrients were filtered through a 0.45-micrometer (μm) capsule filter. Pesticide samples analyzed for pesticide and herbicide compounds were collected from 5 of the 30 wells during 2019 and from 1 well in 2020; pesticide and herbicide compounds frequently detected in previous nationwide studies were targeted for sampling (Barbash and Resek, 1996; Kolpin and others, 1998; Scribner and others, 2007). All of the pesticide samples were filtered through a 47-millimeter (mm) diameter glass fiber filter with a pore size of 0.70 μm. Samples that required refrigeration (nutrients and organic compounds) were kept chilled at 4 degrees Celsius (°C) and shipped chilled to the appropriate laboratories for analyses.
Laboratory Analysis
Groundwater-quality samples were analyzed for major ions, nutrients, and trace elements at the National Water Quality Laboratory (NWQL), in Lakewood, Colorado, and for selected organic compounds at the NWQL or at the Organic Geochemistry Research Laboratory (OGRL), in Lawrence, Kansas. Organic compound samples were submitted to the NWQL for analyses of 81 common pesticides and to the OGRL for analyses of 35 common pesticides. Descriptions of laboratory analytical methods are published in Fishman and Friedman (1989) and Fishman (1993) for major ions analyses; Patton and Kryskalla (2003) for nutrients analyses; and Fishman and Friedman (1989), Struzeski and others (1996), Garbarino (1999), and Garbarino and others (2006) for trace-element analyses. Pesticide analysis was done by solid-phase extraction and gas chromatography/mass spectrometry following the methods published in Zaugg and others (1995), Lindley and others (1996), Sandstrom and others (2001), and Madsen and others (2002).
Concentrations of major ions, nutrients, and trace elements were reported by the NWQL and reviewed for completeness and accuracy. The analytical quantification procedure used by the NWQL for reporting results is based on the long-term method detection level (LT-MDL) and laboratory reporting level (LRL). The LT-MDL acts as a censoring limit for most analytical methods at the NWQL, and its purpose is to limit the false positive rate to less than or equal to 1 percent (Foreman and others, 2021). The LRL is twice the LT-MDL and was established to limit the false negative rate to less than or equal to 1 percent (Foreman and others, 2021). A constituent concentration is considered estimated by the laboratory when results are greater than the LT-MDL and less than the LRL; that is, detection is considered likely, but quantitation is considered estimated. Therefore, a remark code of “E” (estimated) is assigned by the laboratory for these results. Additionally, when measured values were greater than the calibration range of the instrument or when the field measurement stabilization criteria were not met, the data are reported with an “E” remark code (Foreman and others, 2021). In rare instances, a value lower than the LT-MDL might be reported at the discretion of the analyst. In contrast to the LT-MDL and LRL reporting convention used by the NWQL, the OGRL uses one reporting limit for each constituent that is set equal to or greater than the limit of quantitation (Meyer and others, 2009).
Quality-Assurance and Quality-Control Procedures
Quality-control data were collected to assess the variability and bias that may exist within the sample-collection procedures and laboratory analyses (USGS, variously dated). To test for this variability and bias, two field-blank samples, three sequential-replicate samples, and one matrix-spiked sample for organic compounds were collected in conjunction with environmental samples. Both field-blank samples were analyzed for inorganic constituents and organic compounds.
Field-Blank Analyses
Field-blank samples were collected and processed prior to the collection of environmental samples at two well locations to help ensure that equipment cleaning conducted in the field between the collection of samples from different wells was adequate and that the collection, processing, or transporting procedures in the field did not potentially contaminate the environmental samples. In field-blank samples, low concentrations were detected (0.04–0.235 milligrams per liter [mg/L]) for each of the following major ions: calcium, chloride, magnesium, silica, and sulfate. In one field-blank sample, ammonia was detected at 0.02 mg/L. Concentrations in field-blank samples were low (0.079–3.49 micrograms per liter [μg/L]) compared to concentrations in environmental samples for the following trace elements: barium, lithium, molybdenum, strontium, and vanadium. Concentrations of cobalt, copper, manganese, and zinc in field-blank samples (0.123–3.7 μg/L) were similar to the concentrations of these constituents in environmental samples. The cause for the detection of low-level concentrations of some inorganic constituents in the field-blank samples is unknown. To avoid possible bias, values for two constituents (copper and zinc) measured in environmental samples at concentrations similar to those measured in field-blank samples (relative percent differences [RPDs] less than 35 percent) were not included for interpretive purposes. No organic compounds were detected in either of the field-blank samples.
Sequential-Replicate Analyses
Sequential-replicate samples were collected from three different wells to measure the variability in results originating from sampling procedures and analytical methods. An inorganic constituent replicate sample and an organic compound replicate samples were collected together at one well. In addition, another inorganic constituent replicate sample was collected at a second well and another organic compound replicate sample was collected at a third well. To evaluate the potential variability introduced during sample collection, processing, or laboratory analysis, the analytical results measured in an environmental sample were compared with those measured in the associated replicate sample by computing the RPD for each constituent. The RPD between each pair of duplicate samples was calculated by using the following equation:
whereC1
is the constituent concentration measured in the environmental sample, in milligrams per liter or micrograms per liter; and
C2
is the constituent concentration measured in the replicate sample, in milligrams per liter or micrograms per liter.
RPDs of 10 percent or less indicate good agreement between the paired results if the concentrations were sufficiently large compared to their associated LRLs (Foreman and others, 2021; Oden and others, 2011; Mueller and others, 2015). An RPD was not computed if either of the paired results was reported as an estimated concentration or was below the LRL. Most RPDs did not exceed 10 percent for constituents analyzed in the three sequential-replicate samples collected during this study, indicating generally good agreement between the environmental and replicate sample concentrations. RPDs exceeded 10 percent (but were less than 21 percent) for three constituents (orthophosphate, copper, and lead) in samples collected from one of the two wells where inorganic samples were collected. Differences between the concentrations in paired samples where sample concentrations are less than 0.06 mg/L (such as orthophosphate, copper, and lead) can result in large RPD values.
Matrix-Spike Analysis
A spiked environmental sample is an environmental replicate sample to which a known volume containing known concentrations of target constituents is added in the field (Wilde and others, 2002; Martin and others, 2009). Groundwater was collected from the well and processed following standard procedures to produce two samples (USGS, variously dated; Shelton, 1994; Koterba and others, 1995). Spike solution was added to only one of the two groundwater samples, resulting in spiked and unspiked samples (the matrix spike and the “background” sample, respectively). Matrix spikes are usually collected to help determine any matrix interference and the analyzing laboratory’s ability to recover constituent concentrations (Zaugg and others, 1995; Menheer and Brigham, 1997; Mueller and others, 1997). Unspiked and spiked environmental samples were used to assess bias and variability from possible degradation of pesticide constituents resulting in lower concentrations during sample processing, storage, and analysis (Mueller and others, 2015). Analytical recoveries of the spiked target constituents are expressed as percentages of expected (theoretical) concentrations. The percent recoveries of constituents in the spiked environmental sample were compared to those of the laboratory recovery spiked samples to evaluate matrix interferences or degradation of organic compounds. Percent recovery is computed as follows:
whereCspiked
is the measured concentration of the constituent of interest in the spiked environmental sample, in micrograms per liter;
Cunspiked
is the measured concentration of the constituent of interest in the unspiked environmental sample, in micrograms per liter; and
Cexpected
is the theoretical concentration of the constituent of interest in the spiked environmental sample, in micrograms per liter, and is computed as follows:
Csolution
is the concentration of the constituent of interest in the spike solution, in micrograms per liter;
Vspike
is the volume of spike added to the environmental sample, in milliliters; and
Vsample
is the volume of the environmental sample, in liters.
Constituent concentrations less than the LRL in the unspiked sample were set to zero for the purposes of calculating percent recovery. A mixture of target constituents was added to one of the environmental replicate samples for spike analysis. Percent recoveries were computed for each of the constituents, with the optimum value being 100 percent (Mueller and others, 2015; Shoda and others, 2017). Atrazine, deethylatrazine, and tebuthiuron were the only organic compounds detected in environmental samples. Percent recoveries for atrazine and tebuthiuron ranged from 104.8 to 129.6 percent for spiked environmental samples, but percent recovery for deethylatrazine ranged from 69.8 to 82.2 percent. If the percentage recovery for a given constituent in a spiked sample is either high or low, the percentage recovery of the constituent in the corresponding environmental sample results warrant close scrutiny and possible action (Zaugg and others, 1995; Menheer and Brigham, 1997; Mueller and others, 1997; Mueller and others, 2015). Because recoveries for deethylatrazine were consistently low in laboratory recovery spiked samples (less than 82 percent), all concentration results for deethylatrazine detections are reported as estimated. Concentrations of selected organic compounds measured in the unspiked environmental samples that were less than their respective LRLs were considered irrelevant, and their recoveries were not evaluated in the matrix spike results.
Depth to Water and Groundwater Level Changes Since 2012–13
The depth to water was measured prior to lowering the submersible pump into each well for collecting groundwater-quality samples. Depths to water measured during the 2019–20 sampling period ranged from 79.33 ft below land surface at well Li-0613 in Lipscomb County to 504.53 ft below land surface at well Ha-2583 in Hartley County (Ging and Mobley, 2021). Depths to water made in 2019–20 were compared to the measurements collected in 2012–13. All depth to water-level measurements during 2012–13 and 2019 were made in either February or March when irrigation usage is low, whereas the 2020 depth to water-level measurements were made in July when irrigation usage is high.
Depth to water, which was measured from land surface, increased in 28 of the 30 wells with a median difference of 18.17 ft between the 2012–13 and 2019–20 sampling periods (fig. 2; Ging and Mobley, 2021). McGuire (2017) has indicated that groundwater-level declines have occurred throughout the Ogallala aquifer, including its extent in Texas, ever since irrigation became substantial (about 1950). These groundwater-level declines have decreased the amount of groundwater available for use.
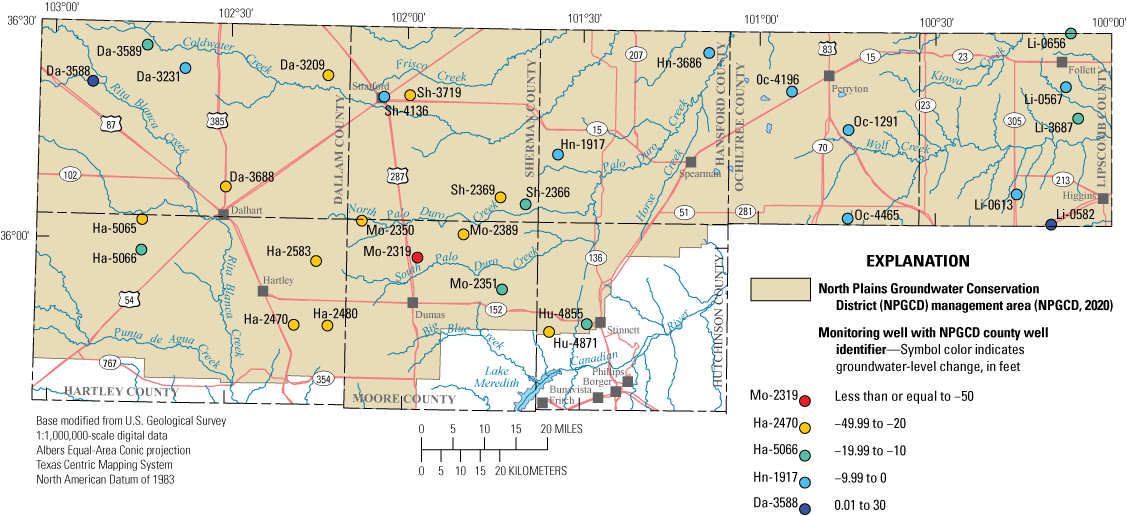
Groundwater-level changes between the 2012–13 and 2019–20 sampling periods at wells completed in the Ogallala aquifer in the Texas Panhandle.
Unexpected decreases in the depth to water of 16.36 ft and 29.62 ft were measured in wells Li-0582 and Da-3588, respectively (fig. 2; Ging and Mobley, 2021), relative to the depth to water measured at these wells during 2012–13. The decreased depth to water in wells Li-0582 and Da-3588 might be related to localized field conditions at the time of sampling. On March 11, 2019, the day when groundwater-level and water-quality data were collected at Li-0582, the lead author of this report heard running water coming from within the well bore, which might indicate water was flowing into the well from a perched groundwater zone. The initial static depth to water from land surface was 201.18 ft, but after the purging process, the depth to water increased to 226.56 ft from land surface. A similar pattern was observed at well Da-3588 where the initial static depth to water was 227.88 ft from land surface, but after the purging process, the depth to water increased to 295.73 ft from land surface. Because the wells have not been pumped for several years prior to the 2019–20 sampling period, the rapid increases in the depth to water at the two wells after purging indicate groundwater levels near these wells can change quickly in response to groundwater withdrawals.
Water-Quality Results and Comparison to 2012–13 Results
Results from the groundwater-quality analyses were compared to the available National Primary Drinking Water Regulations established by the EPA (EPA, 2009) for finished drinking water (hereinafter referred to as the “maximum contaminant levels” or “MCLs”). Results from groundwater-quality analyses were also compared to National Secondary Drinking Water Regulations established by the EPA (secondary drinking-water standards), which are non-enforceable guidelines for finished drinking water, where available (EPA, 2009). In addition to comparing the 2019–20 results from water-quality analyses to selected drinking water standards, the results of water-quality analyses from samples collected in 2019–20 were compared to results from water-quality samples collected during 2012–13.
Hydrochemical Facies and Dissolved-Solids Concentrations
The composition of groundwater and its hydrochemical facies principally are controlled by the composition of recharge water, rock-water interaction, and the mixing of groundwater from different sources (Jones and others, 1997; Elango and Kannan, 2007). The term “hydrochemical facies” refers to a classification scheme used to describe water in terms of the major cation and anion milliequivalents composition. The trilinear diagram (Piper, 1944) is a useful tool for evaluating the relative abundance of major cations and anions and classifying hydrochemical facies or groundwater types, and it was used to evaluate groundwater samples collected from wells completed in the Ogallala aquifer in the Texas Panhandle during 2019–20 (fig. 3). Most of the groundwater samples collected during 2019–20 were classified as magnesium-bicarbonate type groundwater, which is similar to the classification of groundwater in samples collected during the 2012–13 (Baldys and others, 2014). Exceptions to the predominant magnesium-bicarbonate type classifications included two samples classified as sodium-bicarbonate type obtained from wells Da-3588 and Da-3589, the sample classified as sodium-chloride type obtained from well Oc-4465, and the sample classified as a mixed groundwater type obtained from well Hu-4855. Water samples obtained from wells Da-3588, Ds-3589, and Oc-4465 during 2019–20 were assigned the same groundwater types previously assigned when the wells were sampled during 2012–13 (Baldys and others, 2014). Water from well Hu-4855 seemed to have shifted from a calcium-chloride type groundwater during the 2012–13 sampling event to a more mixed water type, which included larger contributions of magnesium and bicarbonate, during the 2019–20 sampling event.

The relative abundance of major cations and anions with classification of water type for groundwater samples collected from wells completed in the Ogallala aquifer in the Texas Panhandle, 2019–20. Labeled samples are discussed in the text.
Dissolved-solids concentration is a measure of the quantity of dissolved constituents in a sample. Sodium and chloride are often the primary constituents that contribute to elevated dissolved-solids concentrations (Hem, 1985). As groundwater levels decline in an aquifer, deeper saline groundwater can move into areas being affected by groundwater withdrawals (Alley and others, 1999). Therefore, increasing sodium and chloride concentrations levels in groundwater can be a cause for concern among well owners. Dissolved-solids concentrations in samples for the wells sampled during the 2019–20 sampling period ranged from 260 to 774 mg/L with a median dissolved-solids concentration of 316 mg/L, which is slightly higher than the median dissolved-solids concentration for the 2012–13 sampling period of 311 mg/L (fig. 4; Ging and Mobley, 2021). Dissolved-solids concentrations in samples at five wells (Hu-4855, Oc-4465, Li-3687, Oc-4196, and Da-3589) were higher than the secondary drinking water standard of 500 mg/L (EPA, 2009) during the 2019–20 sampling period (fig. 4). Dissolved-solids concentrations in samples at four of those five wells were higher than the secondary drinking water standard of 500 mg/L in the 2012–13 sampling period as well (Ging and Mobley, 2021). Sodium concentrations in samples from the wells collected during the 2019–20 sampling period ranged from 7.5 to 200 mg/L with a median sodium concentration of 23.6 mg/L, which is slightly higher than the median sodium concentration of 21.2 mg/L for the 2012–13 sampling period (fig. 5; Ging and Mobley, 2021). Chloride concentrations measured in samples collected during the 2019–20 sampling period ranged from 4.0 to 253 mg/L with the median chloride concentration of 12.8 mg/L, which is slightly higher than the median chloride concentration of 11.6 mg/L for the 2012–13 sampling period (fig. 5; Ging and Mobley, 2021). Accounting for variability with analyses, median major ion concentrations were similar during the 2012–13 and 2019–20 sampling periods.
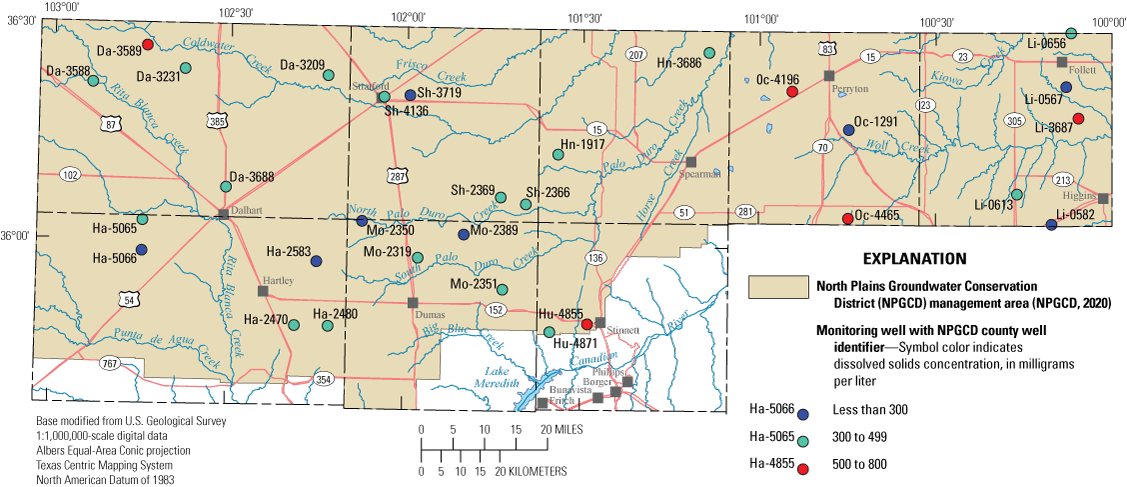
Dissolved-solids concentrations measured in samples collected from wells completed in the Ogallala aquifer in the Texas Panhandle, 2019–20.
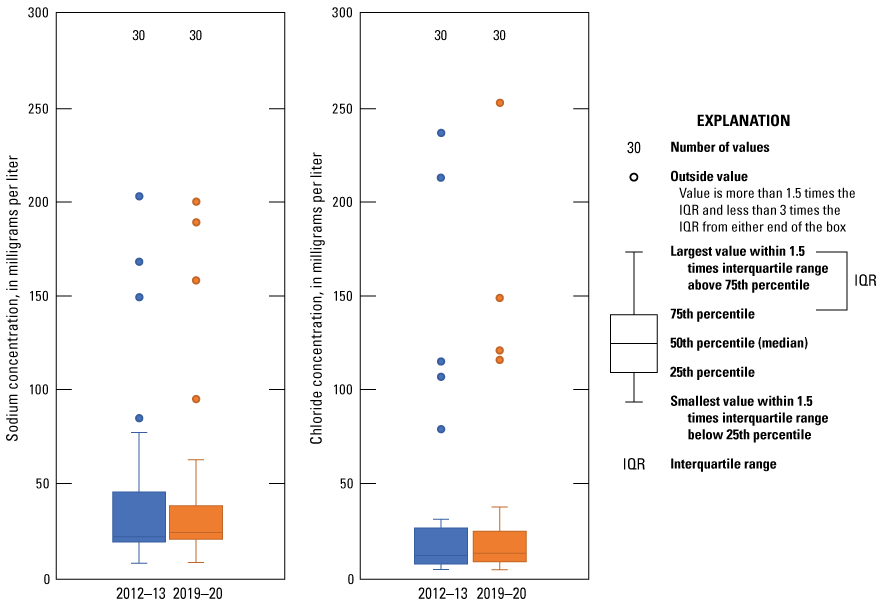
Distribution of sodium and chloride concentrations measured in samples collected from wells completed in the Ogallala aquifer in the Texas Panhandle for the 2012–13 and the 2019–20 sampling periods.
Nutrients
Nitrogen and phosphorus are usually the most commonly detected nutrients when elevated nutrient concentrations are found in groundwater (Ator and Denis, 1997). Ator and Denis (1997, p. 2) explain “although natural sources of nitrogen and phosphorus exist, elevated concentrations of these nutrients in groundwater are typically anthropogenic (human-derived).” Elevated nitrogen and phosphorus concentrations in groundwater frequently result from surface-water infiltration in agricultural or municipal areas where nutrient sources typically include livestock manure, septic systems, wastewater treatment systems, fertilizers, and atmospheric deposition of nitrogen from fossil fuel combustion (Ator and Denis, 1997; Dubrovsky and others, 2010). National background concentrations of nitrate, the most common form of nitrogen in groundwater, are estimated to be 1 mg/L (Dubrovsky and others, 2010). Water with nitrate concentrations higher than 10 mg/L can cause methemoglobinemia (also known as blue-baby syndrome) in infants less than 6 months of age and is considered a major contaminant and threat to groundwater quality in Texas (Chaudhuri and others, 2012). Samples were collected for the determination of four species of nitrogen and one species of phosphorus (orthophosphate). The four species of nitrogen that were analyzed were ammonia, nitrite plus nitrate, nitrite, and total nitrogen. Although organic nitrogen concentrations were not computed for this report, the concentration of organic nitrogen can be estimated by subtracting the sum of ammonia and nitrite plus nitrate from total nitrogen (Patton and Truitt, 2000; Ging and Mobley, 2021). Ammonia concentrations were less than the LRL of 0.010 mg/L for 27 of the 30 wells that were sampled during 2019–20. Nitrite concentrations were less than the LRL of 0.001 mg/L for all wells sampled during 2019–20 (Ging and Mobley, 2021). Nitrate is the dominant nitrogen species in the nitrate plus nitrite analysis because all nitrite concentrations were below the LRL. The median nitrate concentration for the 2019–20 sampling period was 2.25 mg/L, which is similar to the national median nitrate concentration of 2 mg/L determined by Mueller and Helsel (1996). However, nitrate concentrations in samples from two wells (Da-3688 and Sh-4136) exceeded the MCL of 10 mg/L (EPA, 2009) for drinking-water supplies during the 2019–20 sampling period (fig. 6). Wells Da-3688 and Sh-4136 were the same two wells where the nitrate concentrations exceeded 10 mg/L during the 2012–13 sampling period (Baldys and others, 2014). Nitrate concentrations were less than the LRL of 0.08 mg/L for two wells (Da-3588 and Da-3589), which are the only two wells where dissolved oxygen concentrations were less than 1 mg/L (Ging and Mobley, 2021). Total nitrogen concentrations were similar to the nitrate concentrations, indicating that nitrate concentrations account for most of the nitrogen concentrations (Ging and Mobley, 2021). Total nitrogen is defined as the sum of ammonia, nitrite, nitrate, and organic nitrogen (Patton and Truitt, 2000). When comparing median concentrations of nitrate and total nitrogen from the 2012–13 sampling period to the 2019–20 sampling period, median concentrations for both nitrate and total nitrogen increased slightly from the 2012–13 sampling period (2.05 and 2.14 mg/L, respectively) to the 2019–20 sampling period (2.25 and 2.31 mg/L, respectively) (fig. 7). Nitrate concentrations in samples collected from 19 of the 30 wells increased between the two sampling periods, the magnitudes of the increases ranged from 0.01 to 7.9 mg/L. Total nitrogen concentrations in samples collected from 17 of the 30 wells increased between the two sampling periods, and the magnitudes of the increases ranged from 0.01 to 9.4 mg/L (fig. 7; Ging and Mobley, 2021). Accounting for variability with analyses, median concentrations for nutrients species (both nitrogen and phosphorus) were similar for the 2012–13 and 2019–20 sampling periods.
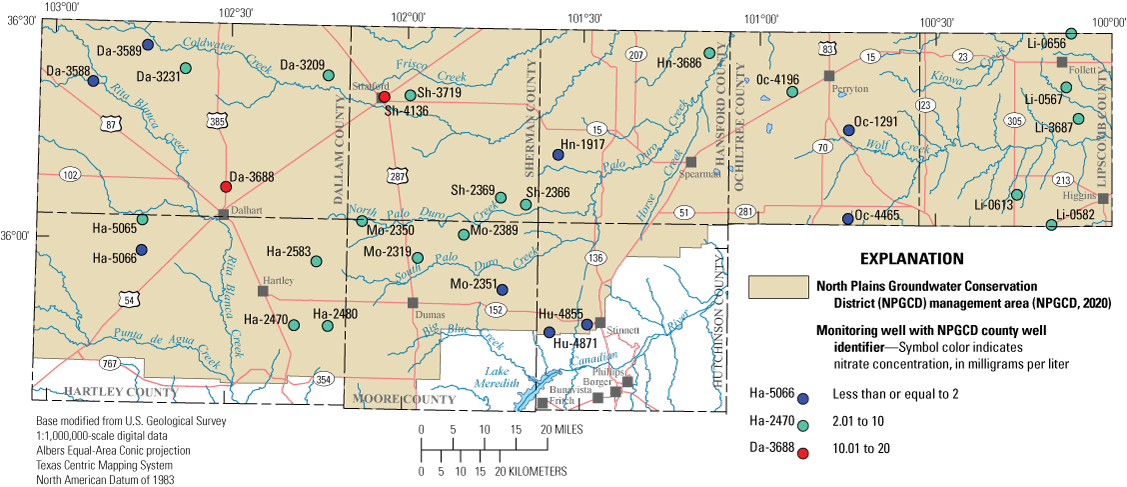
Nitrate concentrations measured in samples collected from wells completed in the Ogallala aquifer in the Texas Panhandle during the 2019–20 synoptic sampling.
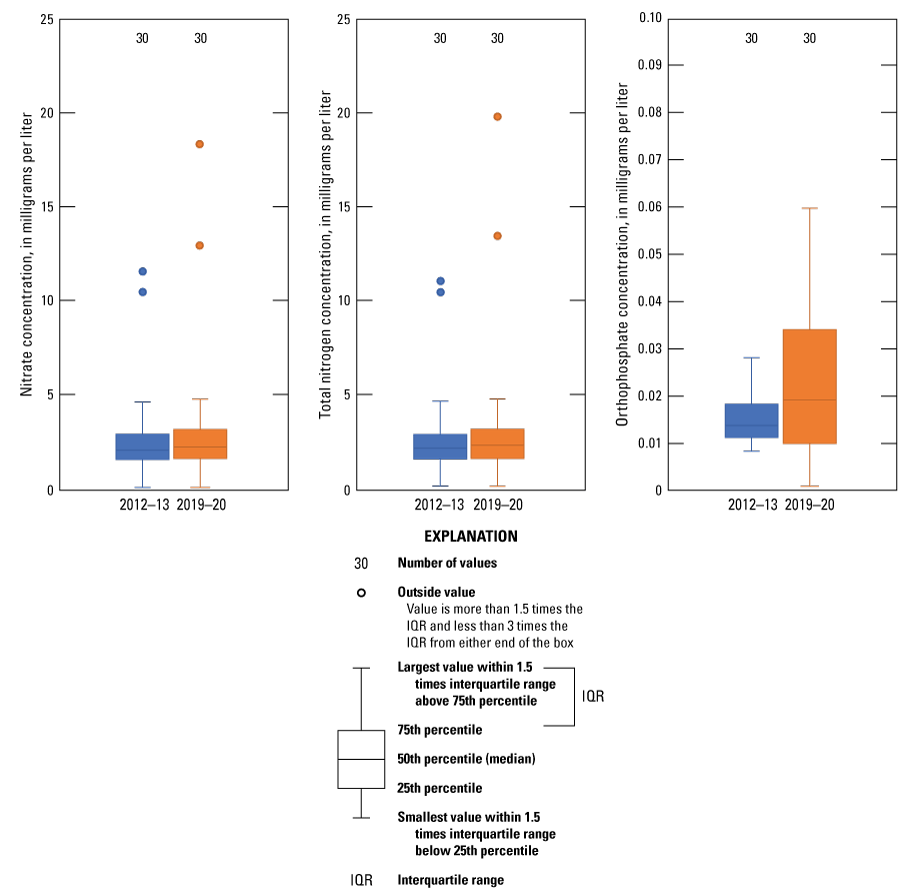
Distribution of nitrate, total nitrogen, and orthophosphate concentrations measured in samples collected from wells completed in the Ogallala aquifer in the Texas Panhandle during the 2012–13 and 2019–20 sampling events.
Phosphorus is important in agricultural systems for the development of high-yielding crops, especially corn, soybeans, and wheat (Domagalski and Johnson, 2012). Long-term over-application of manure and chemical fertilizers that contain phosphorus can lead to phosphorus migration into the groundwater system, resulting in potential contamination of the groundwater resource. In water, phosphorous may be present as orthophosphate (Domagalski and Johnson, 2012). Because the median orthophosphate concentration was less than 0.019 mg/L in samples collected from the 30 wells sampled during 2019–20, it is unlikely that phosphorus contamination of groundwater from agricultural practices is common in the study area. The median orthophosphate concentration in samples collected increased slightly from 0.014 mg/L for the 2012–13 sampling period to 0.019 mg/L for the 2019–20 sampling period (fig. 7).
Trace Elements
Environmental samples from each well were analyzed to determine the concentrations of 23 trace elements, which are substances that typically are present in natural waters at concentrations less than 1.0 mg/L (Hem, 1985). Trace elements are present in small amounts in the environment and can originate from natural and manmade sources (Ayotte and others, 2011). Concentrations of trace elements are more likely to be higher in groundwater than in surface water as a result of rock-water interaction during the movement of groundwater through the rocks that compose the aquifers. Much of the groundwater in the Ogallala aquifer was recharged thousands of years ago (McMahon and others, 2007). Groundwater that has been in an aquifer for a long time can foster extensive rock-water interaction, which can cause trace element concentrations to increase (Elango and Kannan, 2007). Human activities such as fertilizer use, mining activities, and waste disposal also can affect concentrations of trace elements in groundwater (Ayotte and others, 2011). In water-quality samples collected from the 30 wells, concentrations of 16 trace elements were higher than the LRL in at least 50 percent of the samples. MCLs or secondary drinking-water standards are available for only 14 of the 23 trace elements analyzed (table 2). None of the trace element concentrations exceeded their respective MCLs or secondary drinking-water standards (Ging and Mobley, 2021). Median concentrations of trace elements for the 2012–13 sampling period were compared to those for the 2019–20 sampling period for constituents having at least 50 percent of detections in samples above the highest applicable LRL, and variability in analyses was accounted for. Comparison results indicated that median concentrations of two trace elements (lithium and uranium) increased and the median concentrations for two trace elements (barium and molybdenum) decreased (fig. 8). Arsenic concentrations, which can be a concern in the Ogallala aquifer because of agricultural use in the area, were all below the MCL of 10 μg/L in the 30 samples collected from all 30 wells sampled in 2019–20 (fig. 9; Ging and Mobley, 2021). Arsenic can naturally occur in rocks and soils but also was used in certain pesticide applications in the study area (Welch and others 2000; Thelin and Stone, 2010).
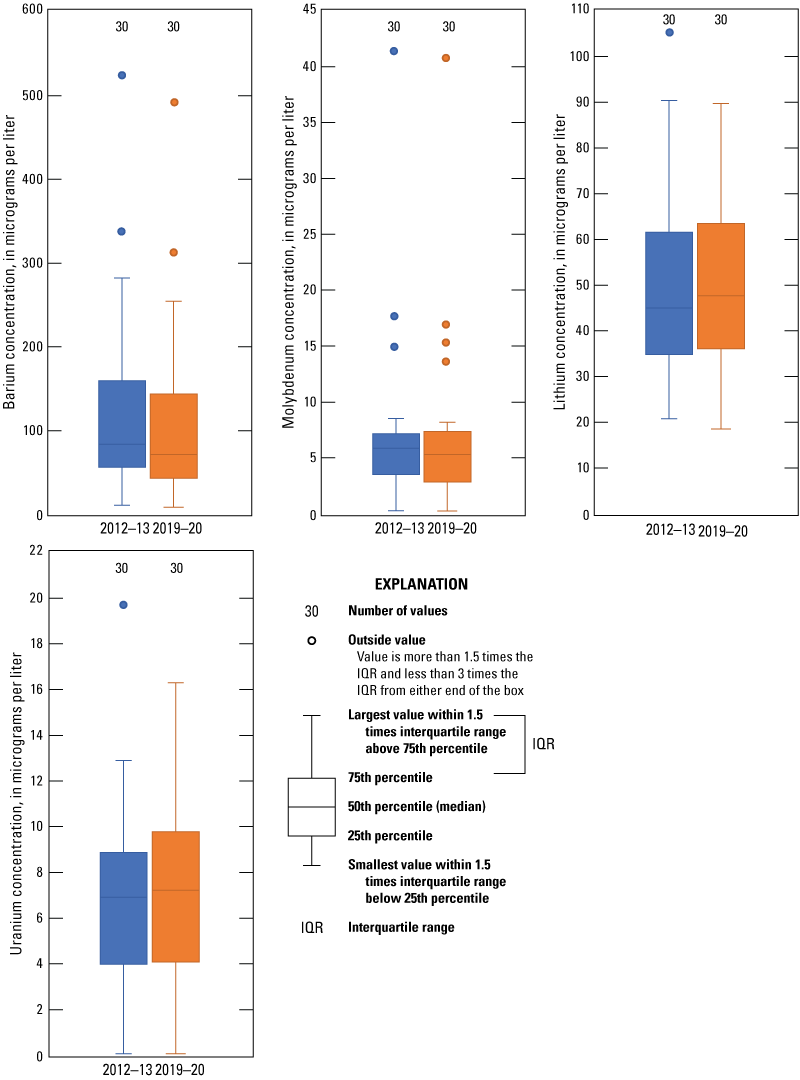
Distribution of barium, molybdenum, lithium, and uranium concentrations measured in samples collected from wells completed in the Ogallala aquifer in the Texas Panhandle during the 2012–13 and the 2019–20 sampling periods.
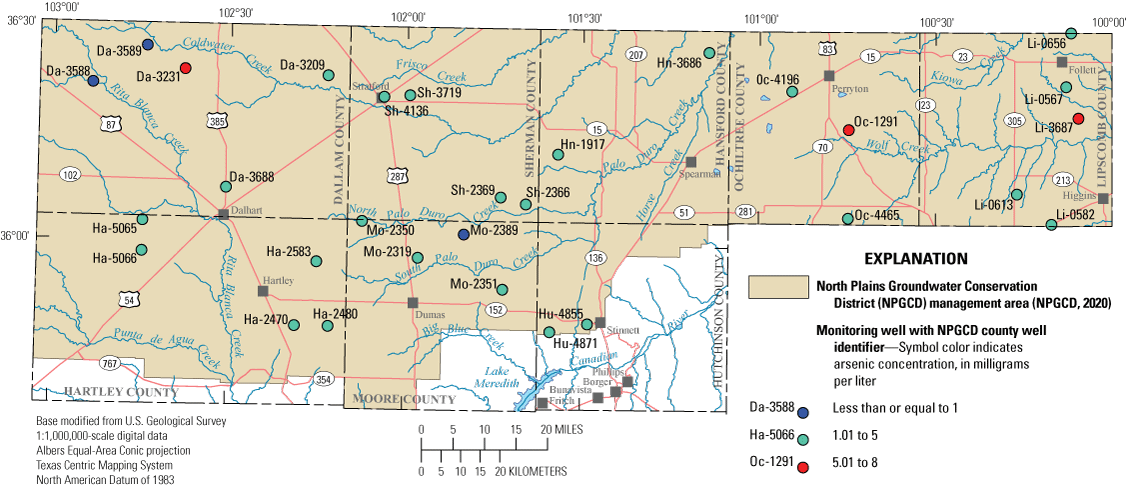
Arsenic concentrations measured in samples collected from wells completed in the Ogallala aquifer in the Texas Panhandle during the 2019–20 synoptic sampling.
Table 2.
National primary (maximum contaminant level) and secondary drinking water standards for the selected trace elements that were analyzed in samples collected during 2019–20.[na, not applicable; EPA, U.S. Environmental Protection Agency]
Organic Compounds
Detections of organic compounds such as pesticides in groundwater samples are potential indicators of anthropogenic effects on groundwater quality. Organic compounds detected in groundwater samples likely originate from surface water, which contains pesticides, seeping into the groundwater in the study area. Groundwater samples were submitted to the NWQL for analyses of 81 common pesticides and to the OGRL for analyses of 35 common pesticides. Samples were collected for organic compound analyses at 6 of the 30 wells sampled in the 2019–20 study (Ging and Mobley, 2021). Organic compounds were detected in samples collected from four of the six wells (Sh-2369, Hn-1917, Ha-2480, and Li-0656) (fig. 10). Atrazine and deethylatrazine were the only organic compounds detected at the four wells. Atrazine was detected in samples from two of the four wells (Hn-1917 and Li-0656) and deethylatrazine was detected in samples from all four wells. Concentrations of atrazine and deethylatrazine detections were all less than 0.05 μg/L (Ging and Mobley, 2021). Atrazine is a triazine herbicide commonly used to control broadleaf and grassy weeds in a variety of crops such as corn and sorghum (EPA, 2021) and has a drinking-water standard of 3.0 µg/L (EPA, 2009). Deethylatrazine is a pesticide degradate of atrazine (EPA, 2006).
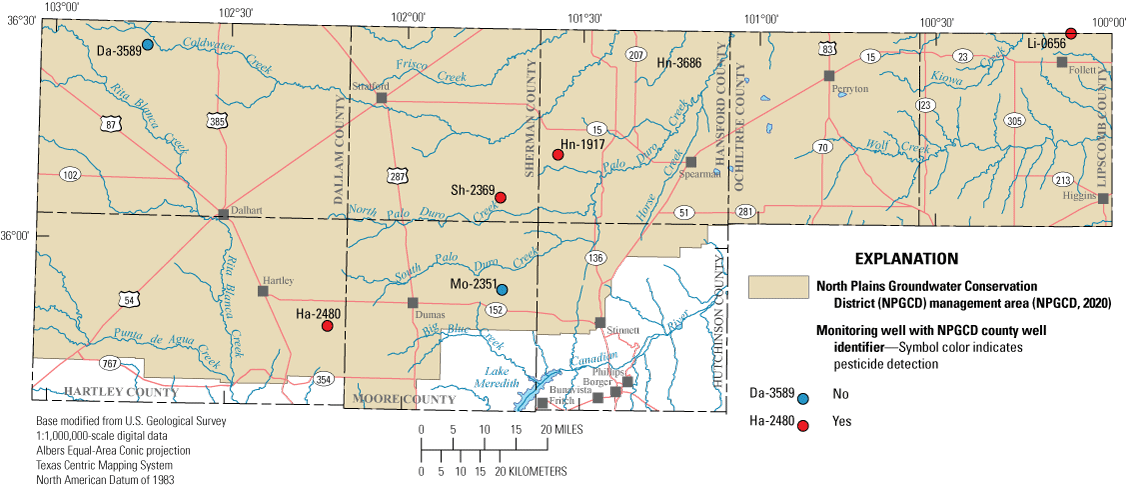
Pesticide detections in samples collected from wells completed in the Ogallala aquifer in the Texas Panhandle during the 2019–20 synoptic sampling.
During the 2012–13 sampling period, samples from the same six wells were analyzed for the same organic compounds at NWQL and OGRL. Three of the four wells where organic compounds were detected in the samples collected during the 2019–20 sampling period were the same wells where organic compounds were detected in the sample collected during the 2012–13 sampling period. Atrazine, deethylatrazine, and tebuthiuron were detected during the 2012–13 sampling period, and concentrations were all less than 0.03 μg/L, which is slightly less than the detected concentrations during the 2019–20 sampling period. Tebuthiuron was not detected during the 2019–20 sampling period (Ging and Mobley, 2021).
Summary
The Ogallala aquifer is part of the High Plains aquifer system, an extensively used groundwater system underlying parts of Colorado, Kansas, Nebraska, New Mexico, Oklahoma, South Dakota, Wyoming, and Texas. The High Plains aquifer system is commonly divided in three parts (northern, central, and southern). The central High Plains aquifer system underlies the North Plains Groundwater Conservation District (NPGCD) in the Texas Panhandle. The study area for this report consists of the five northernmost counties that form the border between Texas and Oklahoma (Dallam, Sherman, Hansford, Ochiltree, and Lipscomb Counties) and parts of Hartley, Moore, and Hutchinson Counties, all of which are contained in the NPGCD management area.
The Ogallala aquifer is the primary source of water for agricultural and municipal purposes in the study area. Because most of the groundwater in the study area is withdrawn from the Ogallala aquifer, information on the quality of groundwater in the Ogallala aquifer is useful for resource characterization. During a previous 2012–13 study, the U.S. Geological Survey in cooperation with the NPGCD, collected and analyzed water-quality samples from 30 groundwater monitoring wells in the Texas Panhandle. The results of this previous study were published in 2014 to help provide an initial characterization of the spatial and temporal variability of water quality in the NPGCD management area. This report documents the results of a followup synoptic sampling study completed between March 2019 and July 2020 by the U.S. Geological Survey, in cooperation with the NPGCD, to further characterize the spatial and temporal characteristics of groundwater in the NPGCD management area. Depth to water measurements and groundwater-quality samples were collected from 30 monitoring wells in the NPGCD management area as part of a second synoptic sampling effort divided between March 2019 and July 2020. Results from the 2019–20 synoptic sampling were compared to drinking-water standards and results from the 2012–13 synoptic sampling for selected constituents. The groundwater-quality data that were collected were assessed in the context of results obtained from quality-control samples collected in conjunction with the environmental samples.
Selected physicochemical properties were measured in the field, and groundwater-quality samples were collected and processed at each of the 30 wells. Physicochemical properties measured onsite were dissolved oxygen, pH, specific conductance, groundwater temperature, turbidity, and alkalinity. Depth to water-level measurements were made prior to collecting groundwater-quality samples, which were analyzed for major ions, nutrients, trace elements, and selected organic compounds. Quality-control samples were collected to assess the variability and bias that may exist within the sample-collection procedures and laboratory analyses.
The depth to water, which was measured from land surface, increased in 28 of the 30 wells with a median difference of 18.17 feet between the 2012–13 and 2019–20 sampling periods. Major ion analyses indicate that most of the groundwater sampled during the 2019–20 sampling period was classified as magnesium-bicarbonate type groundwater, which was similar to the groundwater sampled during the 2012–13 sampling period. Dissolved-solids concentrations for the wells sampled during the 2019–20 sampling period ranged from 260 to 774 milligrams per liter (mg/L) with a median dissolved-solids concentration of 316 mg/L, which is slightly higher than the median dissolved-solids concentration of 311 mg/L for the 2012–13 sampling period. Median sodium and chloride concentrations for wells sampled during the 2019–20 sampling period (23.6 and 12.8 mg/L, respectively) were slightly higher than the median sodium and chloride concentrations for the 2012–13 sampling period (21.2 and 11.6 mg/L, respectively). Accounting for variability with analyses, median major ion concentrations were similar for the 2012–13 and 2019–20 sampling periods.
Of the four nitrogen species analyzed, nitrate is the dominant nitrogen species. The median nitrate concentration for the 2019–20 sampling period was 2.25 mg/L, which is similar to the national median nitrate concentration of 2 mg/L. Total nitrogen concentrations (the sum of ammonia, nitrite, nitrate, and organic nitrogen) were similar to the nitrate concentrations, indicating that nitrate concentrations accounted for most of the total nitrogen concentrations. Both median concentrations for nitrate and total nitrogen increased slightly from the 2012–13 sampling period (2.05 and 2.14 mg/L, respectively) to the 2019–20 sampling period (2.25 and 2.31 mg/L, respectively). Accounting for variability in analyses, median concentrations for nutrients species (both nitrogen and phosphorus) were similar during the 2012–13 and 2019–20 sampling periods.
Of the 23 trace elements analyzed in groundwater from the 30 wells, 16 were detected above the laboratory reporting level in at least 50 percent of the samples. Only 14 of the 23 trace elements have associated maximum contaminant level or secondary drinking-water standards. None of the trace element concentrations exceeded any maximum contaminant level or secondary drinking-water standards. When comparing median concentrations of trace elements from the 2012–13 sampling period to those from the 2019–20 sampling period for constituents with at least 50 percent of samples detected above the higher laboratory reporting level and accounting for variability in analyses, median concentrations of two trace elements (lithium and uranium) increased and the median concentrations for two trace elements (barium and molybdenum) decreased.
Groundwater samples were submitted to the National Water Quality Laboratory for analyses of 81 common pesticides and to the Organic Geochemistry Research Laboratory for analyses of 35 common pesticides. Samples were collected for organic compound analyses at 6 of the 30 wells sampled in the 2019–20 study. Organic compounds were detected in samples collected from four of the six wells. Atrazine and deethylatrazine were the only organic compounds detected. Concentrations of atrazine and deethylatrazine detections were all less than 0.05 micrograms per liter. During the 2012–13 sampling period, the same six wells were analyzed for the same organic compounds at National Water Quality Laboratory and Organic Geochemistry Research Laboratory. Three of the four wells where organic compounds were detected in the samples collected during the 2019–20 sampling period were the same wells where organic compounds were detected in the samples collected during the 2012–13 sampling period. Atrazine, deethylatrazine, and tebuthiuron were detected during the 2012–13 sampling period.
References Cited
Alley, W.M., Reilly, T.E., and Franke, O.L., 1999, Sustainability of ground water resources: U.S. Geological Survey Circular 1186, 79 p., accessed April 30, 2021, at https://doi.org/10.3133/cir1186.
Ator, S.W., and Denis, J.M., 1997, Relation of nitrogen and phosphorus and groundwater the land-use import subunits of the Potomac River basin: U.S. Geological Survey Water-Resources Investigations Report 96–4268, accessed August 27, 2021, at https://doi.org/10.3133/wri964268.
Ayotte, J.D., Gronberg, J.M., and Apodaca, L.E., 2011, Trace elements and radon in groundwater across the United States: U.S. Geological Survey Scientific Investigations Report 2011–5059, 115 p., accessed May 10, 2021, at https://doi.org/10.3133/sir20115059.
Baldys, S., III, Haynie, M.M., and Beussink, A.M., 2014, Water quality of the Ogallala Formation, central High Plains aquifer within the North Plains Groundwater Conservation District, Texas Panhandle, 2012–13: U.S. Geological Survey Scientific Investigations Report 2014–5188, 64 p., accessed May 10, 2021, at https://doi.org/10.3133/sir20145188.
Becker, M.F., Bruce, B.W., Pope, L.M., and Andrews, W.J., 2002, Ground-water quality in the central High Plains aquifer, Colorado, Kansas, New Mexico, Oklahoma, and Texas, 1999: U.S. Geological Survey Water-Resources Investigations Report 02–4112, 64 p., accessed May 10, 2021, at https://doi.org/10.3133/wri20024112.
Chaudhuri, S., Ale, S., DeLaune, P., and Rajan, N., 2012, Spatio-temporal variability of groundwater nitrate concentration in Texas—1960 to 2010: Journal of Environmental Quality, v. 41, no. 6, p. 1806–1817. https://doi.org/10.2134/jeq2012.0022.
Cunningham, W.L., and Schalk, C.W., comps., 2011, Groundwater technical procedures of the U.S. Geological Survey: U.S. Geological Survey Techniques and Methods, book 1, chap. A1, 151 p., accessed May 10, 2021, at https://doi.org/10.3133/tm1A1.
Domagalski, J.L., and Johnson, H., 2012, Phosphorus and groundwater—Establishing links between agricultural use and transport to streams: U.S. Geological Survey Fact Sheet 2012–3004, 4 p., accessed May 10, 2021, at https://doi.org/10.3133/fs20123004.
Dubrovsky, N.M., Burow, K.R., Clark, G.M., Gronberg, J.M., Hamilton, P.A., Hitt, K.J., Mueller, D.K., Munn, M.D., Nolan, B.T., Puckett, L.J., Rupert, M.G., Short, T.M., Spahr, N.E., Sprague, L.A., and Wilber, W.G., 2010, The quality of our Nation’s waters—Nutrients in the Nation’s streams and groundwater, 1992–2004: U.S. Geological Survey Circular 1350, 174 p., accessed May 10, 2021, at https://doi.org/10.3133/cir1350.
Dugan, J.T., McGrath, T.S., and Zelt, R.B., 1994, Water level changes in the High Plains aquifer—Predevelopment to 1992: U.S. Geological Survey Water-Resources Investigations Report 94–4027, 56 p., accessed August 24, 2021, at https://doi.org/10.3133/wri944027.
Elango, L., and Kannan, R., 2007, Rock-water interaction and its control on chemical composition of groundwater: Developments in Environmental Science, v. 5, p. 229–243, accessed August 19, 2021, at https://doi.org/10.1016/S1474-8177(07)05011-5.
Fishman, M.J., ed., 1993, Methods of analysis by the U.S. Geological Survey National Water Quality Laboratory—Determination of inorganic and organic constituents in water and fluvial sediments: U.S. Geological Survey Open File Report 93–125, p. 27–45, accessed May 10, 2021, at https://doi.org/10.3133/ofr93125.
Fishman, M.J., and Friedman, L.C., 1989, Methods for determination of inorganic substances in water and fluvial sediments: U.S. Geological Survey Techniques of Water Resources Investigations, book 5, chap. A1, 545 p., accessed May 10, 2021, at https://doi.org/10.3133/twri05A1.
Foreman, W.T., Williams, T.L., Furlong, E.T., Hemmerle, D.M., Stetson, S.J., Jha, V.K., Noriega, M.C., Decess, J.A., Reed-Parker, C., and Sandstrom, M.W., 2021, Comparison of detection limits estimated using single- and multi-concentration spike-based and blank-based procedures: Talanta, v. 228, article 122139, 15 p., accessed May 10, 2021, at https://doi.org/10.1016/j.talanta.2021.122139.
Garbarino, J.R., 1999, Methods of analysis by the U.S. Geological Survey National Water Quality Laboratory—Determination of dissolved arsenic, boron, lithium, selenium, strontium, thallium, and vanadium using inductively coupled plasma-mass spectrometry: U.S. Geological Survey Open-File Report 99–093, 31 p., accessed May 10, 2021, at https://doi.org/10.3133/ofr9993.
Garbarino, J.R., Kanagy, L.K., and Cree, M.E., 2006, Determination of elements in natural-water, biota, sediment and soil samples using collision/reaction cell inductively coupled plasma-mass spectrometry: U.S. Geological Survey Techniques and Methods, book 5, chap. B1, 88 p., accessed May 10, 2021, at https://doi.org/10.3133/tm5B1.
Ging, P.B., and Mobley, C.A., 2021, Water-quality and depth to water for groundwater wells primarily completed in the Ogallala aquifer within the North Plains Groundwater Conservation District, Texas Panhandle, 2012–13 and 2019–20: U.S. Geological Survey data release, https://doi.org/10.5066/P9J57VYB.
Gutentag, E.D., Heimes, F.J., Krothe, N.C., Luckey, R.R., and Weeks, J.B., 1984, Geohydrology of the High Plains aquifer in parts of Colorado, Kansas, Nebraska, New Mexico, Oklahoma, South Dakota, Texas, and Wyoming: U.S. Geological Survey Professional Paper 1400–B, 63 p., accessed May 10, 2021, at https://doi.org/10.3133/pp1400B.
Hem, J.D., 1985, Study and interpretation of the chemical characteristics of natural water (3d ed.): U.S. Geological Survey Water-Supply Paper 2254, 263 p., accessed May 10, 2021, at https://doi.org/10.3133/wsp2254.
Jones, S.A., Lee, R.W., and Busby, J.F., 1997, Chemical evolution and estimated flow velocity of water in the Trinity aquifer, south-central Texas: U.S. Geological Survey Water-Resources Investigations Report 97–4078, accessed August 27, 2021, at https://doi.org/10.3133/wri974078.
Koterba, M.T., Wilde, F.D., and Lapham, W.W., 1995, Groundwater data-collection protocols and procedures for the National Water-Quality Assessment Program—Collection and documentation of water-quality samples and related data: U.S. Geological Survey Open-File Report 95–399, 113 p., accessed May 10, 2021, at https://doi.org/10.3133/ofr95399.
Madsen, J.E., Sandstrom, M.W., and Zaugg, S.D., 2002, Methods of analysis by the U.S. Geological Survey National Water Quality Laboratory—A method supplement for the determination of fipronil and degradates in water by gas chromatography/mass spectrometry: U.S. Geological Survey Open-File Report 02–462, 11 p., accessed May 10, 2021, at https://doi.org/10.3133/ofr02462.
Martin, J.D., Stone, W.W., Wydoski, D.S., and Sandstrom, M.W., 2009, Adjustment of pesticide concentrations for temporal changes in analytical recovery, 1992–2006: U.S. Geological Survey Scientific Investigations Report 2009–5189, 23 p., 9 app., accessed May 10, 2021, at https://doi.org/10.3133/sir20095189.
McGuire, V.L., 2017, Water-level and recoverable water in storage changes, High Plains aquifer, predevelopment to 2015 and 2013–15: U.S. Geological Survey Scientific Investigations Report 2017–5040, 14 p., accessed on May 11, 2021, at https://doi.org/10.3133/sir20175040.
McMahon, P.B., Böhlke, J.K., and Carney, C.P., 2007, Vertical gradients in water chemistry and age in the northern High Plains aquifer, Nebraska, 2003: U.S. Geological Survey Scientific Investigations Report 2006–5294, 58 p., accessed August 27, 2021, at https://doi.org/10.3133/sir20065294.
Menheer, M.A., and Brigham, M.E., 1997, Ground-water sampling methods and quality-control data for the Red River of the North Basin, Minnesota, North Dakota, and South Dakota, 1993–95: U.S. Geological Survey Water-Resources Investigations Report 96–4317, 34 p., accessed May 10, 2021, at https://doi.org/10.3133/wri964317.
Meyer, M.T., Loftin, K.A., Lee, E.A., Hinshaw, G.H., Dietze, J.E., and Scribner, E.A., 2009, Determination of glyphosate, its degradation product aminomethylphosphonic acid, and glufosinate, in water by isotope dilution and online solid-phase extraction and liquid chromatography/tandem mass spectrometry: U.S. Geological Survey Techniques and Methods, book 5, chap. A10, 32 p., accessed on April 30, 2021, at https://doi.org/10.3133/tm5A10.
Mueller, D.K., and Helsel, D.R., 1996, Nutrients in the Nation’s water—Too much of a good thing?: U.S. Geological Survey Circular 1136, 24 p., accessed May 10, 2021, at https://doi.org/10.3133/cir1136.
Mueller, D.K., Martin, J.D., and Lopes, T.J., 1997, Quality-control design for surface-water sampling in the National Water-Quality Assessment program: U.S. Geological Survey Open-File Report 97–223, 17 p., accessed May 10, 2021, at https://doi.org/10.3133/ofr97223.
Mueller, D.K., Schertz, T.L., Martin, J.D., and Sandstrom, M.W., 2015, Design, analysis, and interpretation of field quality-control data for water-sampling projects: U.S. Geological Survey Techniques and Methods, book 4, chap. C4, 54 p., accessed May 10, 2021, at https://doi.org/10.3133/tm4C4.
National Oceanic and Atmospheric Administration, 2021, National Centers for Environmental Information—Climatic data online search tool: U.S. Department of Commerce, National Climatic Data Center online database, accessed April 30, 2021, at https://www.ncdc.noaa.gov/oa/ncdc.html.
North Plains Groundwater Conservation District [NPGCD], 2020, Hydrology and groundwater resources 2019–2020: Dumas, Tex., North Plains Groundwater Conservation District, 49 p., accessed April 30, 2021, at http://northplainsgcd.org/wp-content/uploads/2020-Hydrology-and-GW-Resources-Double-Side.pdf.
Oden, J.H., Brown, D.W., and Oden, T.D., 2011, Groundwater quality of the Gulf Coast aquifer system, Houston, Texas, 2010: U.S. Geological Survey Data Series 598, 64 p., accessed May 10, 2021, at https://doi.org/10.3133/ds598.
Patton, C.J., and Kryskalla, J.R., 2003, Methods of analysis by the U.S. Geological Survey National Water Quality Laboratory, evaluation of alkaline persulfate digestion as an alternative to Kjeldahl digestion for determination of total and dissolved nitrogen and phosphorus in water: U.S. Geological Survey Water-Resources Investigations Report 03–4174, 33 p., accessed May 10, 2021, at https://doi.org/10.3133/wri034174.
Patton, C.J., and Truitt, E.P., 2000, Methods of analysis by the U.S. Geological Survey National Water Quality Laboratory—Determination of ammonium plus organic nitrogen by a Kjeldahl digestion method and an automated photometric finish that includes digest cleanup by gas diffusion: U.S. Geological Survey Open-File Report 00–170, 31 p., accessed May 10, 2021, at https://doi.org/10.3133/ofr00170.
Ryder, P.D., 1996, Groundwater atlas of the United States—Segment 4, Oklahoma, Texas: U.S. Geological Survey Hydrologic Investigations Atlas 730–E, 30 p., accessed May 10, 2021, at https://doi.org/10.3133/ha730E.
Sandstrom, M.W., Stroppel, M.E., Foreman, W.T., and Schroeder, M.P., 2001, Methods of analysis by the U.S. Geological Survey National Water Quality Laboratory—Determination of moderate-use pesticides and selected degradates in water by C-18 solid-phase extraction and gas chromatography/mass spectrometry: U.S. Geological Survey Water-Resources Investigations Report 01–4098, 70 p., accessed May 10, 2021, at https://doi.org/10.3133/wri20014098.
Scanlon, B.R., Dutton, A., and Sophocleous, M., 2002, Groundwater recharge in Texas: Texas Water Development Board contract report no. 2000483340, 84 p., accessed August 24, 2021, at https://www.twdb.texas.gov/publications/reports/contracted_reports/doc/2000483340.pdf.
Scribner, E.A., Battaglin, W.A., Gilliom, R.J., and Meyer, M.T., 2007, Concentrations of glyphosate, its degradation product, aminomethylphosphonic acid, and glufinosate in ground- and surface-water, rainfall, and soil samples collected in the United States, 2001–06: U.S. Geological Survey Scientific Investigations Report 2007–5122, 111 p., accessed September 1, 2021, at https://doi.org/10.3133/sir20075122.
Shelton, L.R., 1994, Field guide for collecting and processing stream-water samples for the National Water-Quality Assessment Program: U.S. Geological Survey Open-File Report 94–455, 42 p., accessed May 10, 2021, at https://doi.org/10.3133/ofr94455.
Shoda, M.E., Nowell, L.H., Bexfield, L.M., Sandstrom, M.W., and Stone, W.W., 2017, Recovery data for surface water, groundwater and lab reagent samples analyzed by the USGS National Water Quality Laboratory schedule 2437, water years 2013–15: U.S. Geological Survey data release, accessed May 10, 2021, at https://doi.org/10.5066/F7QZ28G4.
Struzeski, T.M., DeGiacomo, W.J., and Zayhowski, E.J., 1996, Methods of analysis by the U.S. Geological Survey National Water Quality Laboratory—Determination of dissolved aluminum and boron in water by inductively coupled plasma-atomic emission spectrometry: U.S. Geological Survey Open-File Report 96–149, 17 p., accessed May 10, 2021, at https://doi.org/10.3133/ofr96149.
Thelin, G.P., and Stone, W.W., 2010, Method for estimating annual atrazine use for counties in the conterminous United States, 1992–2007: U.S. Geological Survey Scientific Investigations Report 2010–5034, 129 p., accessed May 11, 2021, at https://doi.org/10.3133/sir20105034.
U.S. Environmental Protection Agency [EPA], 2006, Decision documents for atrazine: U.S. Environmental Protection Agency web page, accessed September 1, 2021, at https://www3.epa.gov/pesticides/chem_search/reg_actions/reregistration/red_PC-080803_1-Apr-06.pdf.
U.S. Environmental Protection Agency [EPA], 2009, National primary drinking water regulations: U.S. Environmental Protection Agency report, EPA 816–F–09–004, 7 p., accessed May 10, 2021, at https://www.epa.gov/sites/production/files/2016-06/documents/npwdr_complete_table.pdf.
U.S. Environmental Protection Agency [EPA], 2021, Environmental topics—Atrazine: U.S. Environmental Protection Agency web page, accessed September 1, 2021, at https://www.epa.gov/ingredients-used-pesticide-products/atrazine.
U.S. Geological Survey [USGS], 2021, USGS water data for the Nation: U.S. Geological Survey National Water Information System database, accessed May 10, 2021, at https://doi.org/10.5066/F7P55KJN.
U.S. Geological Survey [USGS], [variously dated], National field manual for the collection of water-quality data: U.S. Geological Survey Techniques of Water-Resources Investigations, book 9, chaps. A1–A9. [Also available at https://www.usgs.gov/mission-areas/water-resources/science/national-field-manual-collection-water-quality-data-nfm?qt-science_center_objects=0#qt-scie nce_center_objects.]
Welch, A.H., Watkins, S.A., Helsel, D.R., and Focazio, M.J., 2000, Arsenic in ground-water resources of the United States: U.S. Geological Survey Fact Sheet 063–00, 4 p., accessed September 1, 2021, at https://doi.org/10.3133/fs06300.
Wilde, F.D., Radtke, D.B., Gibs, J., and Iwatsubo, R.T., eds., 2002, Processing of water samples (ver. 2.2, 2019): U.S. Geological Survey Techniques of Water-Resources Investigations, book 9, chap. A5, accessed May 10, 2021, at https://doi.org/10.3133/twri09A5.
Zaugg, S.D., Sandstrom, M.W., Smith, S.G., and Fehlberg, K.M., 1995, Methods of analysis by the U.S. Geological Survey National Water Quality Laboratory—Determination of pesticides in water by C-18 solid-phase extraction and capillary-column gas chromatography/mass spectrometry with selected-ion monitoring: U.S. Geological Survey Open-File Report 95–181, 60 p., accessed May 10, 2021, at https://doi.org/10.3133/ofr95181.
Conversion Factors
Datum
Vertical coordinate information is referenced to the North American Vertical Datum of 1988 (NAVD 88).
Horizontal coordinate information is referenced to the North American Datum of 1983 (NAD 83).
Supplemental Information
Concentrations of chemical constituents in groundwater are given in either milligrams per liter (mg/L) or micrograms per liter (µg/L).
Abbreviations
EPA
U.S. Environmental Protection Agency
LRL
laboratory reporting level
LT-MDL
long-term method detection level
MCL
maximum contaminant level
NPGCD
North Plains Groundwater Conservation District
NWQL
National Water Quality Laboratory
OGRL
Organic Geochemistry Research Laboratory
RPD
relative percent difference
USGS
U.S. Geological Survey
For more information about this publication, contact
Director, Oklahoma-Texas Water Science Center
U.S. Geological Survey
1505 Ferguson Lane
Austin, TX 78754-4501
For additional information, visit
https://www.usgs.gov/centers/ot-water
Publishing support provided by
Lafayette Publishing Service Center
Disclaimers
Any use of trade, firm, or product names is for descriptive purposes only and does not imply endorsement by the U.S. Government.
Although this information product, for the most part, is in the public domain, it also may contain copyrighted materials as noted in the text. Permission to reproduce copyrighted items must be secured from the copyright owner.
Suggested Citation
Mobley, C.A., and Ging, P.B., 2022, Depth to water and water quality in groundwater wells in the Ogallala aquifer within the North Plains Groundwater Conservation District, Texas Panhandle, 2019–20, and comparison to 2012–13 conditions: U.S. Geological Survey Scientific Investigations Report 2022–5026, 25 p., https://doi.org/10.3133/sir20225026.
ISSN: 2328-0328 (online)
Study Area
Publication type | Report |
---|---|
Publication Subtype | USGS Numbered Series |
Title | Depth to water and water quality in groundwater wells in the Ogallala aquifer within the North Plains Groundwater Conservation District, Texas Panhandle, 2019–20, and comparison to 2012–13 conditions |
Series title | Scientific Investigations Report |
Series number | 2022-5026 |
DOI | 10.3133/sir20225026 |
Year Published | 2022 |
Language | English |
Publisher | U.S. Geological Survey |
Publisher location | Reston, VA |
Contributing office(s) | Oklahoma-Texas Water Science Center |
Description | Report: vii, 25 p.; Data release; Dataset |
Country | United States |
State | Texas |
Other Geospatial | Ogallala aquifer |
Online Only (Y/N) | Y |
Google Analytic Metrics | Metrics page |