Groundwater Quality Near the Montebello Oil Field, Los Angeles County, California
Links
- Document: Report (5.8 MB pdf) , HTML , XML
- Data Release: USGS data release — Water chemistry data for samples collected at groundwater sites in the Montebello Oil Field study area, September 2014–October 2018, Los Angeles County, California
- Download citation as: RIS | Dublin Core
Acknowledgments
This study was funded by the California State Water Resources Control Board Oil and Gas Regional Monitoring Program and with additional U.S. Geological Survey Cooperative Matching Funds.
We are grateful to the well owners who granted the U.S. Geological Survey permission to sample their wells. We would also like to thank the peer reviewers whose comments improved this manuscript.
Abstract
Groundwater quality and potential sources and migration pathways of chemical constituents associated with hydrocarbon-bearing formations were assessed by the U.S. Geological Survey for the California State Water Resources Control Board Oil and Gas Regional Monitoring Program (RMP). Groundwater samples were collected as part of the RMP from 21 preexisting wells used for public supply, monitoring, or irrigation in and near the Montebello Oil Field and analyzed for constituents associated with hydrocarbon-bearing formations and constituents used to identify recently recharged groundwater and older groundwater. The newly collected RMP data were supplemented with historical sample data from 849 groundwater wells and analyzed with respect to explanatory factors that have the potential to influence water quality. Potential sources and migration pathways of fluids (water, gas, or oil) from hydrocarbon-bearing formations that could affect groundwater quality in the Montebello Oil Field include large volumes of recycled produced water (water withdrawn from an oil well and brought to the surface that may include oil, water, and gas from the geologic formation and water or gas injected for enhanced recovery) that have been reinjected since the 1960s to enhance oil production, oil and gas wells with well-integrity issues, and oil and gas wells with an uncemented annulus that intersects groundwater resource zones.
Trace amounts of dissolved petroleum hydrocarbons, thermogenic gas (propane through pentane range), or both, were detected in seven groundwater samples collected in 2014 and 2018 as part of the RMP. Five of those samples also contained manufactured volatile organic compounds and at least some modern-age groundwater (recharged during or after 1953), indicating that the hydrocarbons could have originated from surficial, or shallow, sources unrelated to oil and gas development. Two samples contained low concentrations of petroleum hydrocarbons (less than 0.1 microgram per liter) and did not contain detections of manufactured volatile organic compounds in pre-modern groundwater. These samples were collected from relatively deep wells (greater than 140 meters below land surface) with perforations completed in marine sediments that may contain water with similar compositions to produced water.
The RMP sample results and available historical data in and near the Montebello Oil Field did not provide conclusive evidence that oil and gas development has adversely affected groundwater resources. All samples with detectable petroleum hydrocarbons, thermogenic gases, or both, were collected from sites that also are within 500 meters of anthropogenic hydrocarbon sources not associated with oil and gas development or sources. In addition, naturally occurring sources of hydrocarbons that exist at intervals shallower than, or are in areas outside of, economically productive oil- and gas-producing zones could affect groundwater quality.
A definitive analysis of relations of groundwater quality to potential anthropogenic and natural explanatory factors was not possible because of the low density of new and historical sampling data, particularly in parts of the Montebello Oil Field where the largest relative risks to groundwater from hydrocarbon-bearing formations exist. Areas to consider for more detailed monitoring and analysis in the future that may present the largest relative potential risks to groundwater quality include (1) areas downgradient from historical surface ponds and sumps and (2) areas with co-located high net injection (oil reservoir injection exceeds production), old oil and gas wells that may be more likely to develop well-integrity issues than newer wells, and oil and gas wells with uncemented boreholes intersecting groundwater zones. To help fill gaps resulting from sparse groundwater wells, temperature and resistivity borehole log data could be analyzed to locate anomalies that identify potential areas where relatively warm or saline water from deeper hydrocarbon-bearing formations is present in groundwater.
Introduction
Groundwater is a critical resource in California and provides a large percentage of the State’s total freshwater supply, particularly in dry years when surface water is less plentiful. Protecting this supply is essential for human health, food production, and the economy. California is also rich in energy resources. California is the seventh largest producer, by volume, of crude oil in the United States (U.S. Energy Information Administration, 2020) with almost 500 active or inactive onshore oil fields. Some of this development is stratigraphically and geographically near groundwater resources used for the State’s water supply (Davis and others, 2018a). Formations containing groundwater used for supply typically are separated from hydrocarbon-bearing formations by geologic layers with low permeability. Potential pathways for migration of fluids from hydrocarbon-bearing formations to groundwater resources include spills or disposal at the land surface and leaking well bores and casings (Richter and Kreitler, 1991; Darrah and others, 2014; Hammond and others, 2020). Natural geologic pathways such as faults and discontinuous low permeability confining layers also can permit fluid movement between formations (Eichhubl and Boles, 2000; Jung and others, 2015). Small amounts of oil and gas are naturally present in some aquifers that supply drinking water in California. For example, oil and gas shows (appendix 1) in aquifer sediments stratigraphically above oil producing formations were identified at the Oxnard Oil Field in Ventura County (not shown; Rosecrans and others, 2021). In addition, several historical surface oil seeps have been documented in southern Los Angeles County (Hodgson, 1987; Hornafius and others, 1999) and could intersect groundwater. Groundwater quality can be affected by upward movement of poor-quality water from deep hydrocarbon-bearing formations without oil and gas production as a result of groundwater withdrawals from supply wells with perforations in close vertical proximity to deep formations, such as in the Pleasant Valley Groundwater Basin in Ventura County (not shown; Izbicki and others, 2005a, b; United Water Conservation District, 2021).
Large volumes of water are co-produced with hydrocarbons in most geologic settings in California. This produced water (water withdrawn from an oil well and brought to the surface that may include oil, water, and gas from the geologic formation and water or gas injected for enhanced recovery) typically contains relatively large amounts of dissolved salts and other potential contaminants that make the water unsuitable for human consumption or other uses; therefore, the water must be recycled or disposed (Orem and others, 2014; Blondes and others, 2017; McMahon and others, 2018). Produced water primarily has been either discharged to lined and unlined surface disposal ponds to be evaporated or reinjected into deep geologic formations. Historical contamination of soils and shallow groundwater in parts of California and elsewhere has been linked to disposal of produced water into ponds (California Department of Water Resources, 1961a; Entrix, Inc., 2009; Brantley and others, 2014). Injected water can be beneficially used for subsidence control and enhanced oil recovery (EOR) techniques, such as water flooding, steam flooding, and cyclic steam flooding. However, underground migration of water from oil-bearing formations is potentially enhanced by increased fluid pressures caused by water injection activities (Gillespie and others, 2019).
Hydraulic fracturing is an EOR technique of injecting water and chemicals to increase the permeability and yield of hydrocarbons to oil and gas wells in low permeability sediments. The process has potential to affect groundwater resources from fracturing fluid spills, leaks from wells with integrity issues or inadequate annular cement seal, improperly plugged and abandoned wells, improper treatment or disposal of wastewater and fracturing fluids, or creation of fractures in shallow formations (within 200 meters [m] of groundwater; Jackson and others, 2013; Vengosh and others, 2014; U.S. Environmental Protection Agency, 2016). However, hydraulic fracturing is used in a small number of oil fields in California, primarily in the San Joaquin Basin (not shown), and involves much smaller volumes of injected water than more widely used EOR techniques (California Council on Science and Technology, 2015; McMahon and others, 2018).
The California State Water Resources Control Board (State Water Board) implemented the Oil and Gas Regional Monitoring Program (RMP) in 2015 to assess effects of oil and gas development on groundwater designated for any beneficial use as required by California Senate Bill 4 (SB4 statutes of 2013). The RMP assesses potential groundwater-quality effects associated with EOR (water flooding, steam flooding, and hydraulic fracturing), underground injection of oil field wastewater, produced water disposal at the land surface, and geologic or natural sources of chemical constituents (California State Water Resources Control Board, 2019a). The U.S. Geological Survey (USGS) is the technical lead in conducting the RMP through the California Oil, Gas, and Groundwater (COGG) Program, working in cooperation with the State Water Board and in partnership with other State and local agencies. The RMP design includes (1) assessing the location of groundwater resources with less than 10,000 milligrams per liter (mg/L) total dissolved solids, which is the upper limit for potential sources of drinking water (U.S. Environmental Protection Agency, 2019), in proximity to oil and gas fields; (2) assessing evidence for the presence or absence of constituents potentially derived from hydrocarbon-bearing formations in groundwater, and if present, identifying potential migration pathways or processes that may be responsible; and (3) determining how oil and gas development has affected groundwater quality relative to other processes (U.S. Geological Survey, 2019).
As part of the RMP, groundwater near the Montebello Oil Field, located in Los Angeles County, California (fig. 1), was selected by the State Water Board (in consultation with the Los Angeles Regional Water Quality Control Board) as an assessment priority. Relative to the approximately 500 onshore oil fields in California, the Montebello Oil Field has a high volume of water injected into hydrocarbon-bearing formations, a high density of oil and gas wells, a moderate vertical proximity of oil and gas development to groundwater resources, and a moderate density of overlying water wells (Davis and others, 2018a). Additionally, the Montebello Oil Field is proximal to substantial natural and enhanced regional groundwater recharge areas for the Coastal Plain of Los Angeles (herein referred to as the Los Angeles Basin), where groundwater wells supply water to meet the needs of millions of people (Reichard and others, 2003; Edwards and others, 2009; California Department of Water Resources, 2015).
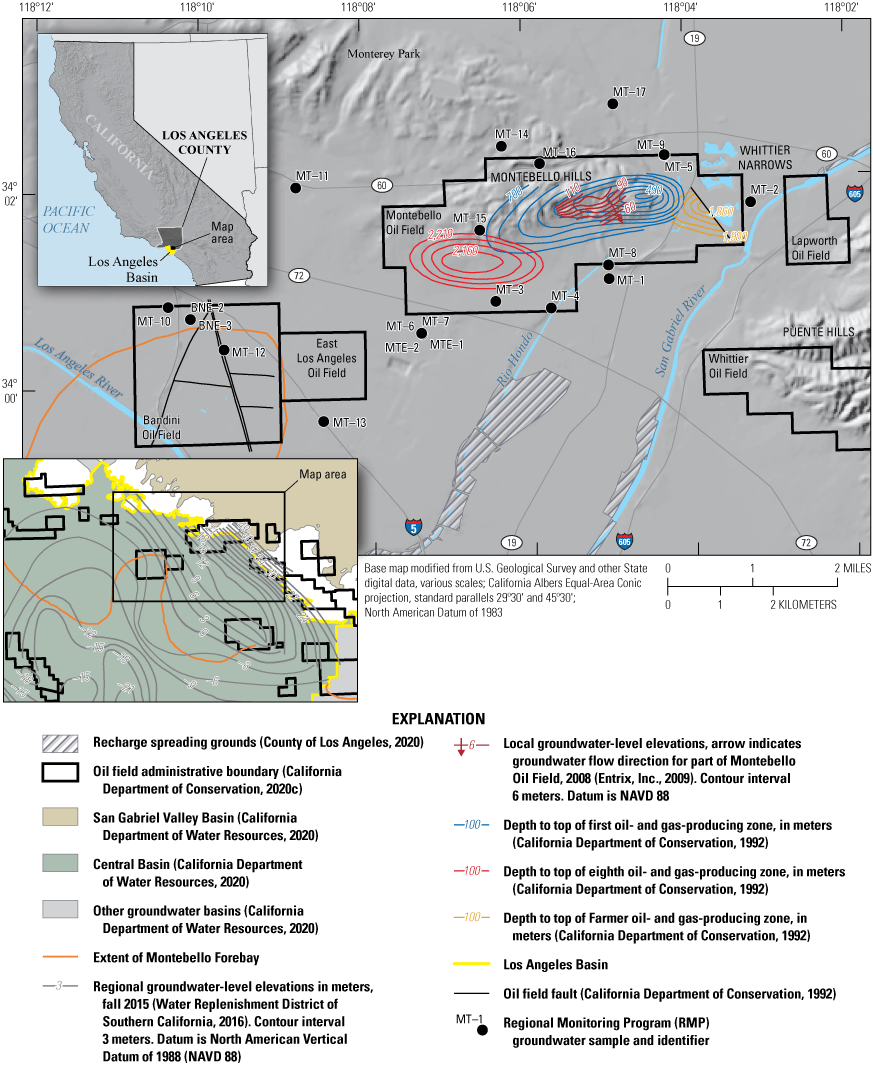
Montebello Oil Field study area and Regional Monitoring Program groundwater sample sites (U.S. Geological Survey, 2021), Los Angeles County, California, 2014 and 2017–18.
Factors specific to the Montebello Oil Field that could increase the risk for subsurface migration of fluids from hydrocarbon-bearing formations include large volumes of recycled produced water that have been reinjected since the 1960s to enhance oil production (California Department of Conservation, variously dated; California Department of Conservation, 1992; Davis and others, 2018a), old oil and gas wells that may be more likely to develop well-integrity issues than newer wells, and oil and gas wells with an uncemented annulus that intersects groundwater zones. Groundwater quality also can be affected by surficial features such as disposal ponds (used for evaporation of produced water), well sumps (used to dispose of drilling mud, cuttings, or fluids), and other infrastructure at the land surface (Entrix, Inc., 2009). Produced water disposal via injection wells can be a risk factor in some oil fields but is not a common practice in the Montebello Oil Field.
Evaluating Groundwater Quality
This study was designed to identify evidence of fluids from oil and gas development in groundwater and the sources and migration pathways responsible for the presence of those fluids, including those not associated with oil and gas development. The study approach consisted of the following components:
-
Groundwater chemistry and isotope data were obtained from samples collected for the RMP study and supplemented with historical groundwater chemistry data from other USGS and State sources.
-
Available data for potential explanatory factors, including sources and migration pathways of fluids associated with hydrocarbon-bearing formations, were compiled and reviewed to determine fluid migration risk factors that could affect groundwater quality in and near the Montebello Oil Field.
-
Groundwater quality was characterized with respect to chemical constituents associated with hydrocarbon-bearing formations and to determine groundwater ages. General geochemistry patterns were compared with explanatory factors, and concentrations of chemical constituents were presented in relation to water-quality benchmarks to provide context for the quality of groundwater in the study area.
-
The RMP groundwater samples that contained chemical constituents associated with hydrocarbon-bearing formations were assessed in more detail with respect to potential explanatory factors.
-
Study limitations and areas to consider for more detailed monitoring and analysis for the largest relative potential risks to groundwater quality were identified.
Purpose and Scope
This report describes the quality of groundwater and discusses possible sources and migration pathways for the chemical constituents identified in samples that are potentially associated with hydrocarbon-bearing formations in and near the Montebello Oil Field, including formations used for commercial oil and gas production and naturally occurring oil and gas shows in overlying sediments. Groundwater samples were collected during 2014–18 from 21 preexisting monitoring, supply, and irrigation wells with completion depths ranging from 28 to 424 m below land surface (bls). Water samples were analyzed for a wide range of chemical compounds and isotopes to identify those potentially associated with fluids from hydrocarbon-bearing formations, including inorganic elements; nutrients; volatile and semi-volatile organic compounds; dissolved organic carbon and its optical properties; hydrogen and oxygen stable isotopes of water; isotopes of strontium (87Sr/86Sr); radium-224, radium-226, and radium-228; groundwater age-dating tracers (tritium [3H], sulfur hexafluoride [SF6], and carbon-14 in dissolved inorganic carbon [14C-DIC]); dissolved noble gases; light hydrocarbon gases (methane through hexane); carbon stable isotopes in dissolved inorganic carbon; and hydrogen and carbon stable isotopes of methane.
The primary constituents discussed in this report are those with results most helpful for identifying potential effects of oil and gas development on groundwater in and near the Montebello Oil Field: inorganic elements, volatile organic compounds (VOCs), groundwater age-dating tracers (3H and 14C-DIC), light hydrocarbon gases (referred to as gases herein), and isotopes of methane. Additionally, oil companies were invited to share relevant information for inclusion in the study and select oil and gas wells, injectate sites, and groundwater wells for sampling. U.S. Geological Survey scientists also consulted with the California Department of Conservation Division of Geologic Energy Management (CalGEM) and Los Angeles Regional Water Quality Control Board staff that were familiar with the oil field. The goal of these compilation efforts and consultations was to obtain the most complete record of information possible.
Contents of this report focus on groundwater chemistry results from the RMP sampling events completed between 2014 and 2018 and available historical data inside or within 4.8 kilometers (km) of the Montebello Oil Field administrative boundary (referred to as the Montebello Oil Field study area hereinafter) and potential factors that may explain or have the potential to influence groundwater quality, including measures of oil development density, intensity, and proximity; water production and injection volumes; vertical fluid pressure differences; occurrence of oil and gas shows in sediments above oil- and gas-producing zones (zones from which commercial quantities of oil and gas are extracted; appendix 1); oil and gas well construction and integrity; other anthropogenic sources of analyzed constituents; and directions of groundwater flow. A 4.8-km buffer of the administrative boundary was selected as the boundary of the study area to include the estimated potential area of influence from oil and gas development in the Montebello Oil Field.
Study results are presented in three sections. The “Factors That Could Affect Groundwater Quality” section includes an analysis of the potential explanatory factors that represent sources and migration pathways of chemical constituents associated with hydrocarbon-bearing formations. The general distribution of groundwater quality across the study area is presented in the “Groundwater Quality near the Montebello Oil Field” section. The “Potential Sources and Migration Pathways of Chemical Constituents” section includes a detailed discussion of the RMP samples that contained chemical constituents associated with hydrocarbon-bearing formations. These analyses are intended to help improve understanding of how or if oil and gas development and naturally occurring sources of petroleum hydrocarbons outside of production zones have influenced groundwater quality in the Montebello Oil Field study area. Limitations for fully characterizing risk factors are presented in the “Study Limitations and Additional Analysis” section. See appendix 1 and figure 1.1 for definitions and diagram of the oil field features and chemical constituent sources used in the report.
Study-Area Description
The Montebello Oil Field covers approximately 15 square km in southern Los Angeles County, in an area of faulting (Wright, 1991; Saucedo and others, 2000; California Public Utilities Commission, 2001; Reichard and others, 2003). The Montebello Oil Field is situated on the edge of the Montebello Forebay (fig. 1), an unconfined part of the Central Basin that serves as the most important recharge zone for the downgradient confined groundwater aquifers of the Los Angeles Basin that supply water to millions of people (California Department of Water Resources, 1961b; Shelton and others, 2001; Reichard and others, 2003; Edwards and others, 2009). The Montebello Oil Field is in an urban setting dominated by commercial, industrial, and high-density residential land uses (Falcone, 2015) that have potential to affect groundwater quality (Shelton and others, 2001). Before urban development, the land cover of the area included cactus gardens, grass, and agricultural crops, such as oats, fruits, and vegetables (Karubian, 1939) and had been used for cattle grazing (Entrix, Inc., 2009).
Hydrogeologic Setting
Important hydrogeologic features in the area include the Montebello Hills, an east to west ridge in the Montebello Oil Field, and the Whittier Narrows, a narrow, alluvial-filled gap cut between the Montebello and Puente Hills by the San Gabriel River that runs along the eastern side of the field (fig. 1). The Montebello Hills are the surficial expression of anticline structures that trapped the oil and gas reserves in the oil field. Water-bearing sediments underlying the Montebello Hills are shallow (as little as 4 m bls) and minimally productive (Entrix, Inc., 2009). Shallow groundwater in the hills is frequently in discontinuous perched zones that are sometimes affected by confining conditions (Entrix, Inc., 2009). Locally, some of the limited shallow groundwater flows south-southeast toward the thick (greater than 670 m) valley alluvium in the Whittier Narrows adjacent to the Montebello Hills (fig. 1; Entrix, Inc., 2009), where water is used for supply. The study area has more than 180 public-supply wells, and about half of those wells are downgradient from the Montebello Oil Field.
Regional groundwater flow is to the west and southwest (fig. 1; Reichard and others, 2003; Ponti and others, 2014; Water Replenishment District of Southern California, 2016). Whittier Narrows is a 2.4-km gap that provides a natural conduit for groundwater and surface water to move from the upgradient San Gabriel Valley Basin to the Central Basin (fig. 1; U.S. Environmental Protection Agency, 2011). Inflow from the San Gabriel Valley Basin provides most of the natural groundwater replenishment for the Central Basin (California Department of Water Resources, 2004). In addition, artificially enhanced recharge to the alluvial valley aquifer beneath the San Gabriel River for downgradient water supply in Los Angeles groundwater basins has been active since the 1950s (Water Replenishment District of Southern California, 2019a), and two large managed recharge areas are immediately downstream from the Montebello Oil Field (fig. 1). Artificial recharge water has primarily been composed of imported water from the Colorado River (not shown) and northern California via the State Water Project, recycled water that has gone through tertiary treatment, and stormwater (Water Replenishment District of Southern California, 2019a). As of 2019, local stormwater and recycled water became the sole sources of supplemental recharge (Water Replenishment District of Southern California, 2020). Groundwater affected by artificial aquifer recharge in the Montebello Forebay tends to have modern (post-1952) groundwater ages, and detections of anthropogenic VOCs from surface or atmospheric sources are common (Shelton and others, 2001; Reichard and others, 2003).
Oil Field Geology and History
The Montebello Oil Field was discovered in 1917 and quickly became one of the primary oil-producing fields in California (McLaughlin, 1918; Save The Montebello Hills Task Force, 2011). The field consists of eight producing zones in the early Pliocene Repetto and late Miocene Puente Formations (fig. 2; California Department of Conservation, 1992). Zones one through three, the main area production zones (fig. 2), are the shallowest (averaging about 670–1,400 m bls) and produce oil from the Repetto Formation; these zones generally have had the largest volume of oil production since 1977 (fig. 3A). Zones four through eight (about 1,500–2,300 m bls), in the west area, were discovered in the 1930s and have produced oil and gas from the Repetto and Puente Formations. The east area production zones (Upper Nutt, Lower Nutt, Farmer, Cruz, and Baldwin) have produced very little oil or gas (fig. 3A). The oil- and gas-producing zones are overlain by late Pliocene siltstones, early Pleistocene conglomerates and siltstones, and late Pleistocene alluvial fans (Quarles, 1941).
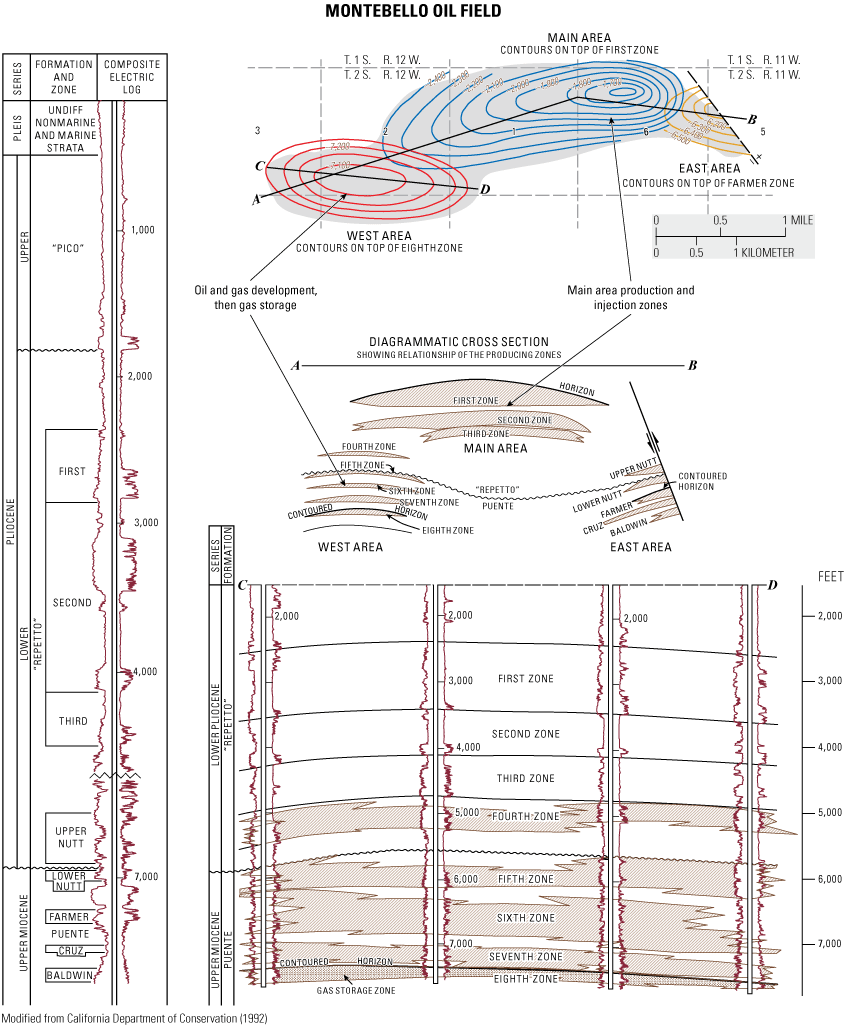
Oil and gas zones, in feet below land surface, Montebello Oil Field, Los Angeles County, California (modified from California Department of Conservation, 1992).
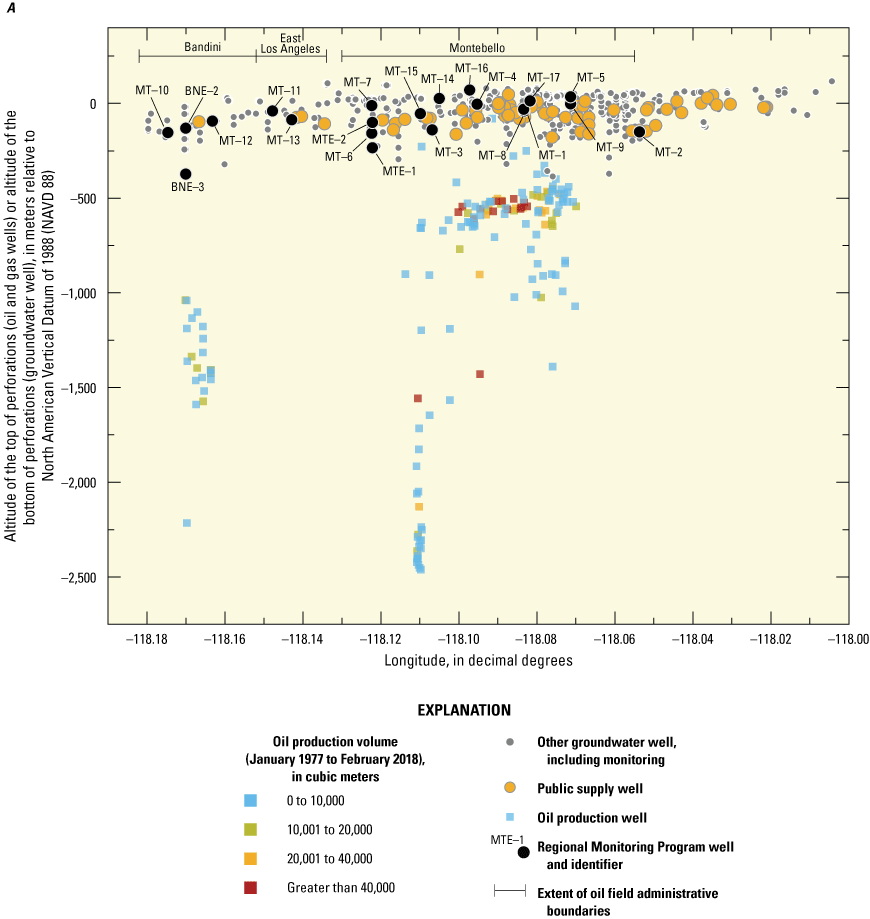
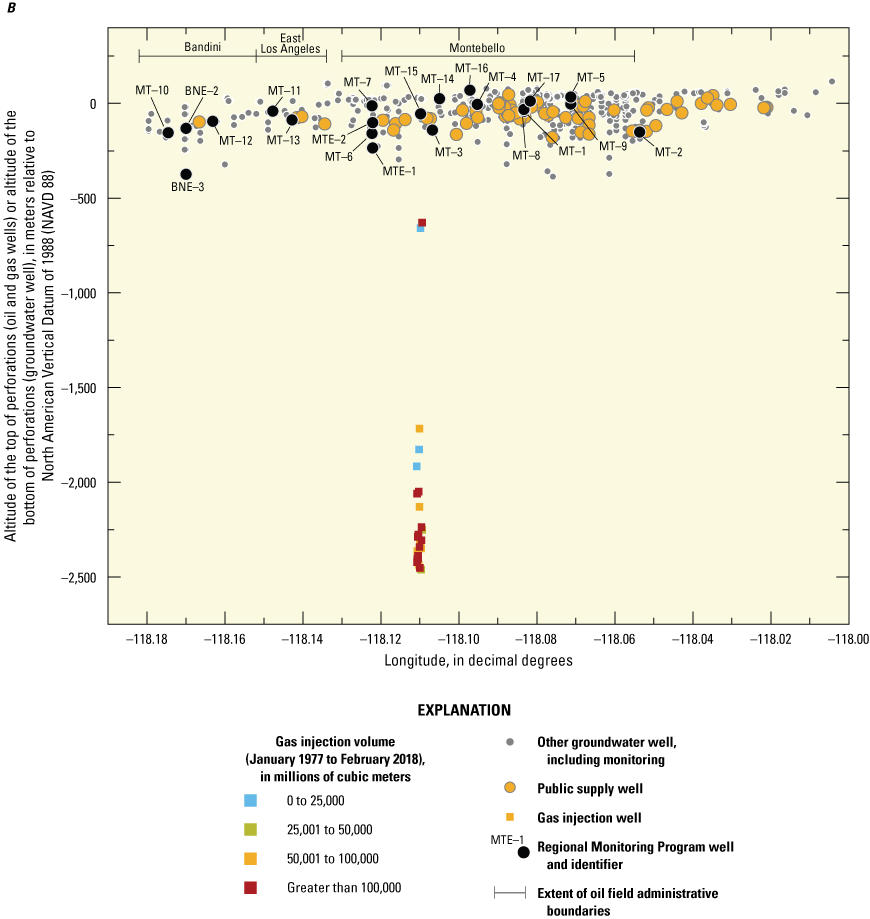
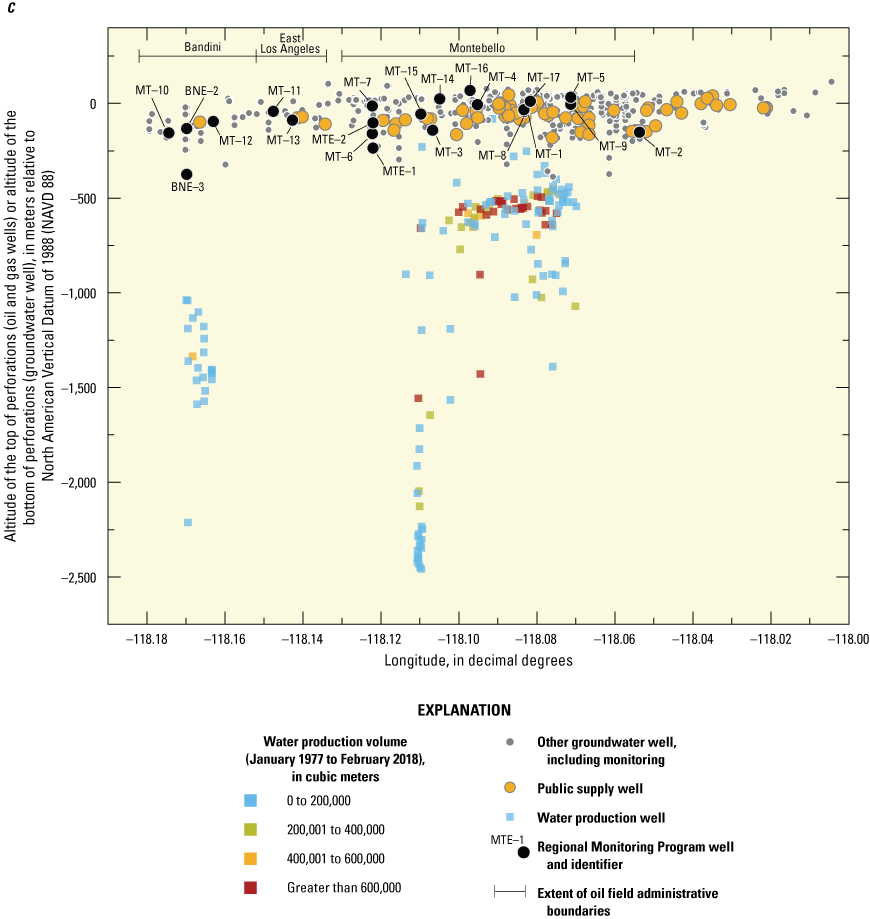
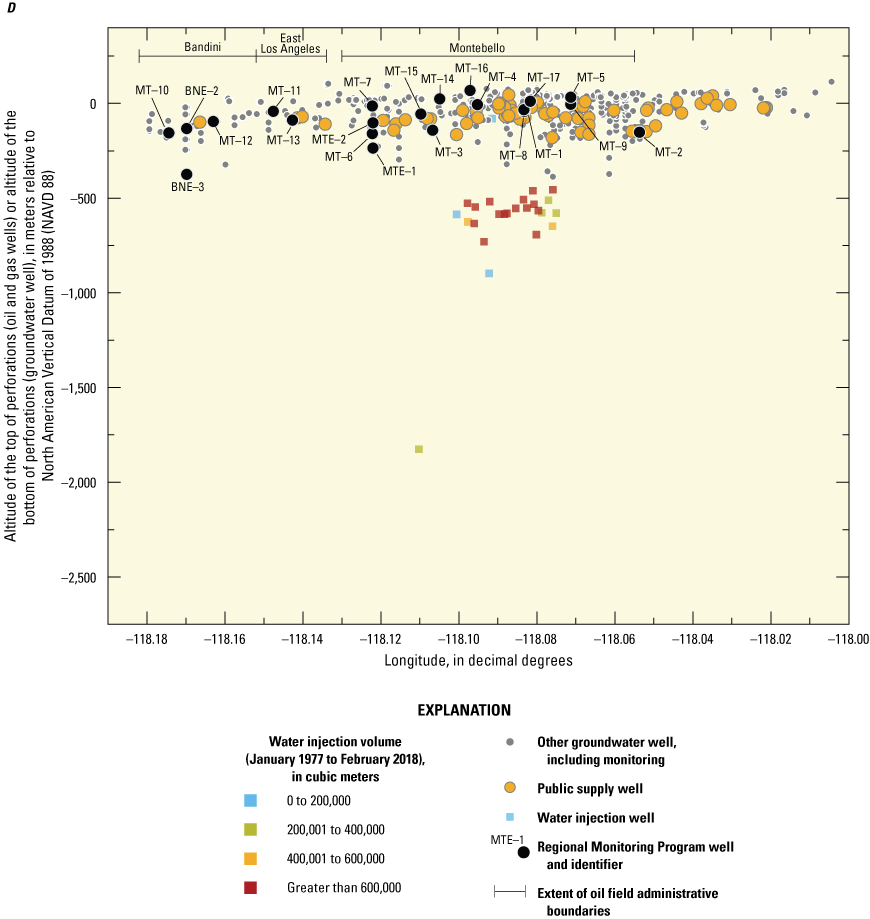
Aggregated plot of longitude versus vertical separation between the depths of Regional Monitoring Program and historical groundwater samples and A, oil production volumes; B, gas injection volumes; C, water production volumes; and D, water injection volumes (California Department of Conservation, 2020c), Montebello Oil Field study area, Los Angeles County, California. Volumes are only shown if well construction information was obtained.
As of 2018, the Montebello Oil Field had 832 (208 active, 20 idle, and 604 abandoned) wells (California Department of Conservation, 2018). The field produced more than 33 million cubic meters (m3) of oil from 1917 to 2018, mostly before 1960 (California Department of Conservation, variously dated). Peak oil production was in 1919. Thermogenic gas, methane and heavier gases produced by the action of high heat and pressure on buried organic matter at depth (appendix 1), was produced from the deeper hydrocarbon-bearing formations of the Montebello Oil Field until formation pressures were reduced. More than 14 million m3 of thermogenic gas was extracted from 1929 to 2018, with almost 11 million m3 of the volume originating as gas that was injected for storage between 1956 and 1996 (California Department of Conservation, variously dated). Starting in 1977, the eighth zone (about 2,300–2,326 m bls) has been used to store thermogenic gas imported from the central United States (fig. 3B; California Department of Conservation, 1992; California Public Utilities Commission, 2001). Imported thermogenic methane gas has been distinguished from locally produced gas in the Montebello Oil Field by the presence of elevated helium concentrations in the imported gas (California Public Utilities Commission, 2001). About 105 million m3 of water was injected into producing zones of the Montebello Oil Field between 1956 and 2017, mostly to enhance oil recovery in the main area (fig. 2; California Department of Conservation, variously dated). Water injection and production volumes (figs. 3C, 3D) are discussed in more detail in the “Potential Subsurface Sources and Migration Pathways” section of this report. Hydraulic fracturing and acidizing practices are rarely used to enhance oil recovery in the Montebello Oil Field. As of July 2014, only 2 percent of the active wells in the Montebello Oil Field had been hydraulically fractured (California Council on Science and Technology, 2015), and hydraulic fracturing was not reported during 2014–21 (California Department of Conservation, 2021).
Other oil fields near the Montebello Oil Field are the Bandini, East Los Angeles, Whittier, and Lapworth Oil Fields (fig. 1). These fields had fewer wells installed and produced less oil and gas during 1977–2018 compared to the Montebello Oil Field (California Department of Conservation, 2020c). The main oil- and gas-producing zones of those fields also are in the Repetto and Puente Formations, and average depths to the oil-producing zones range from 275 to 2,600 m bls (California Department of Conservation, 1992). An additional 334 oil and gas wells (41 active, 20 idle, and 276 plugged or buried) are in other oil fields in the Montebello Oil Field study area. As of 2018, most of those active and idle wells were in the Whittier Oil Field southeast of the Montebello Oil Field (California Department of Conservation, 2018).
Data Used for Analyses
Data used for analyses consisted of groundwater chemistry obtained from samples collected for the RMP study, historical groundwater and oil-field water chemistry data compiled from other sources, and information about the hydrogeologic characteristics and anthropogenic activities that can affect water quality (potential explanatory factors).
Sample Collection and Analysis
Groundwater samples were collected in 2014, 2017, and 2018 from 21 preexisting monitoring, public-supply, and irrigation wells (table 1). Groundwater samples from four monitoring wells were collected downgradient from the Montebello Oil Field in 2014 as part of the exploratory phase of the RMP; those sites were sampled to assess the effectiveness of chemical, isotopic, and groundwater-age tracers for identifying potential sources of salinity, methane, petroleum hydrocarbons, and other constituents in groundwater in selected oil-producing areas of California (McMahon and others, 2017). Groundwater samples were collected from eight monitoring wells, eight public-supply wells, and one inactive irrigation well in 2017 and 2018. For those samples, potential well locations in the Montebello Oil Field study area were selected based on specific criteria: (1) areal and vertical position along groundwater-flow paths, with priority given to locations with shallow and deep well pairs; (2) proximity of wells to current (2018) and historical oil and gas development such as active and abandoned oil and gas wells, produced water injection, and disposal ponds and sumps; and (3) availability of historical water-quality data.
Table 1.
Well construction for groundwater wells sampled as part of the Regional Monitoring Program in the Montebello Oil Field study area, Los Angeles County, California, 2014 and 2017–18 (U.S. Geological Survey, 2021).[U.S. Geological Survey, 2021. Abbreviations: USGS, U.S. Geological Survey; mm/dd/yyyy, month/day/year; NAVD 88, North American Vertical Datum of 1988]
Sample identifier (fig. 1) |
USGS site identification number |
Sample date (mm/dd/yyyy) |
Well type |
Well-perforation interval, meters below land surface |
Depth zone(s) of well-perforation interval |
Length of well perforation, meters |
Land-surface elevation, meters relative to NAVD 88 |
Water-level elevation, meters relative to NAVD 88 |
Dominant depositional environment of sediments at well perforation depths1 |
---|---|---|---|---|---|---|---|---|---|
MTE-1 | 340027118071901 | 09/22/2014 | Monitoring | 274–293 | Intermediate | 18 | 58 | 19 | Marine |
MTE-2 | 340027118071903 | 09/22/2014 | Monitoring | 152–158 | Intermediate | 6 | 58 | 17 | Nonmarine |
BNE-2 | 340040118100904 | 09/23/2014 | Monitoring | 174–180 | Intermediate | 6 | 49 | −5 | Nonmarine |
BNE-3 | 340040118100901 | 09/23/2014 | Monitoring | 405–424 | Deep | 18 | 49 | 11 | Marine |
MT-1 | 340100118045701 | 12/18/2017 | Public supply | 53–96 | Shallow | 43 | 56 | 38 | Marine |
MT-2 | 340148118030901 | 12/19/2017 | Public supply | 51–217 | Shallow and intermediate | 166 | 65 | Unknown | Nonmarine and marine |
MT-3 | 340048118062501 | 12/20/2017 | Public supply | 85–201 | Shallow and intermediate | 116 | 62 | 10 | Marine |
MT-4 | 340047118054601 | 12/20/2017 | Public supply | 37–60 | Shallow | 23 | 55 | 19 | Nonmarine |
MT-5 | 340215118041602 | 01/31/2018 | Monitoring | 25–28 | Shallow | 3 | 63 | 51 | Nonmarine |
MT-6 | 340027118071902 | 01/29/2018 | Monitoring | 210–216 | Intermediate | 6 | 58 | 16 | Marine |
MT-7 | 340027118071905 | 01/30/2018 | Monitoring | 64–70 | Shallow | 6 | 58 | 16 | Nonmarine |
MT-8 | 340108118050001 | 02/28/2018 | Monitoring | 87–90 | Shallow | 3 | 59 | 39 | Marine |
MT-9 | 340215118041601 | 03/01/2018 | Monitoring | 64–67 | Shallow | 3 | 63 | 50 | Nonmarine |
MT-10 | 340047118102801 | 04/11/2018 | Public supply | 94–207 | Shallow and intermediate | 113 | 52 | −5 | Nonmarine |
MT-11 | 340159118085201 | 04/11/2018 | Public supply | 86–118 | Shallow | 32 | 78 | 0 | Nonmarine |
MT-12 | 340021118094701 | 04/12/2018 | Public supply | 101–138 | Shallow and intermediate | 37 | 45 | −2 | Nonmarine |
MT-13 | 335938118083201 | 04/12/2018 | Public supply | 107–134 | Shallow and intermediate | 27 | 47 | 6 | Nonmarine |
MT-14 | 340222118061801 | 10/01/2018 | Monitoring | 81–90 | Shallow | 9 | 116 | 98 | Marine |
MT-15 | 340131118063501 | 10/02/2018 | Monitoring | 148–163 | Intermediate | 15 | 109 | 87 | Marine |
MT-16 | 340211118055001 | 10/03/2018 | Monitoring | 47–53 | Shallow | 6 | 123 | 93 | Marine |
MT-17 | 340247118045401 | 10/04/2018 | Irrigation (unused) | <56–56 | Shallow | Unknown | 70 | 46 | Nonmarine |
Depositional environments based on a three-dimensional chronostratigraphic model developed by Ponti and others (2014).
Maximum well-perforation depths for the 21 sampled wells ranged from 28 to 424 m bls (table 1). Most wells were less than 200 m deep and had well-perforation intervals that corresponded with aquifers used for public supply. Wells were categorized into shallow (less than or equal to 122 m bls), intermediate (122–305 m bls), and deep groundwater zones (greater than 305 m bls). The depth of 122 m bls was chosen as a break point between shallow and intermediate groundwater that could be used for supply; the depth of 305 m bls was chosen as a break point between groundwater that could be used for supply and deeper groundwater not likely used for supply. Samples from public-supply wells were collected before treatment and do not represent water delivered for consumption.
Groundwater samples were processed onsite in a mobile laboratory using procedures designed to minimize changes to the water-sample chemistry or introduction of target analytes during sample collection (U.S. Geological Survey, variously dated). Water was pumped from monitoring wells with a portable stainless-steel submersible pump. Water collected from all sampled wells was delivered from the well to the mobile laboratory through Teflon tubing with stainless-steel connections. Before sample collection, water was flushed from the wells by purging at least three casing volumes. Precleaned bottles were then filled in an enclosed chamber to prevent sample contamination and preserved by chilling, filtration, or chemical treatment as needed to prevent sample degradation. Specific conductance, pH, water temperature, turbidity, and dissolved oxygen were monitored throughout the duration of pumping to assess the stability of the pumped water (U.S. Geological Survey, variously dated). Blank, replicate, and matrix-spike samples were collected to evaluate the reliability of sample processing and analytical methods (appendix 2).
Groundwater samples were analyzed for a wide range of dissolved chemical, gas, and isotopic constituents to identify potential indicators of fluids from hydrocarbon-bearing formations. Where these potential indicators were detected in groundwater, an attempt was made to evaluate available information to ascertain potential sources and migration pathways of those constituents. Chemical constituents analyzed included inorganic elements; nutrients; volatile and semi-volatile organic compounds; dissolved organic carbon and its optical properties; hydrogen and oxygen stable isotopes of water; isotopes of strontium (87Sr/86Sr); radium-224, radium-226, and radium-228; groundwater age-dating tracers (3H, SF6, and 14C-DIC); dissolved noble gases; light hydrocarbon gases (methane through hexane); carbon stable isotopes in dissolved inorganic carbon; and hydrogen and carbon stable isotopes of methane. The primary constituents discussed in this report are those with results most helpful for identifying potential effects of oil and gas development on groundwater in the Montebello Oil Field study area (inorganic elements, VOCs, groundwater age-dating tracers [3H and 14C-DIC], light hydrocarbon gases [referred to as gases herein], and isotopes of methane). Results and analytical methods for these analyses are available in an associated data release (Rodriguez and others, 2022) and in Dillon and others (2016).
For purposes of this report, the term “laboratory detection level” (LDL) is generally defined as the smallest concentration reported by the laboratory as a numeric value. Concentrations smaller than the LDL are typically coded as “less than (<)” the LDL. However, VOCs often are reported as numeric values that are less than the LDL because they are analyzed by an information-rich technique (Rodriguez and others, 2022). A detailed description of LDLs is provided in U.S. Geological Survey (2015).
The groundwater-age tracers 3H and 14C-DIC were used to classify samples as modern (recharged during or after 1953), pre-modern (pre-1953), or a mixture of both. Tritium (3H) is a naturally occurring, radioactive isotope of hydrogen that decays to helium-3 (3He) by beta-decay with a half-life of 12.32 years (Lucas and Unterweger, 2000). This characteristic makes tritium a conservative tracer for defining modern-age waters with detectable 3H. The 3H atom is incorporated in the water molecule in the atmosphere and is introduced and isolated to the groundwater-flow system during recharge. With time, the 3H decays to 3He, which resides as a helium component of the dissolved gas composition of the groundwater. Atmospherically derived carbon-14 (14C) is incorporated into the groundwater’s dissolved inorganic carbon (DIC) component and can be used qualitatively or quantitively to evaluate time since isolation from the atmosphere (Plummer and Sprinkle, 2001; Jurgens and others, 2010; de Jong and others, 2020). Carbon-14 in DIC has a half-life of 5,730 years and is used to date groundwater on the scale of 1,000 to tens of thousands of years before present. Geochemical processes other than radioactive decay act to dilute the atmospheric contribution of 14C required for age dating. Carbon mass and isotope exchange between DIC, soil carbon dioxide (CO2), and aquifer carbonate rock and oxidation of organic carbon are expected to be the primary processes affecting DIC from the sample.
For this study, pre-modern groundwater is defined as having a 3H concentration less than 0.2 tritium units (TU) and a 14C-DIC concentration less than 75 percent modern carbon (pmc; Plummer and Sprinkle, 2001; McMahon and others, 2017). Modern groundwater is defined as having a 3H concentration greater than 1 TU and 14C-DIC greater than 90 pmc (Jurgens and others, 2010; Kulongoski and others, 2010; McMahon and others, 2017). Any concentrations outside of those ranges are defined as a mixture of modern and pre-modern groundwater. These assumed thresholds have been used in other California groundwater studies and represent approximate values for the purposes of classifying groundwater ages and qualitatively assessing relations of groundwater age to concentrations of selected chemical constituents.
Historical Groundwater Chemistry Data
Historical groundwater chemistry data available from other sources were used to supplement data collected as part of the RMP. More than 20,000 groundwater samples have been collected from 849 sites in the Montebello Oil Field study area since 1940 (fig. 4). About 80 percent of those wells have well perforation or total well depth information that can be used to associate chemical properties with depth intervals. Well depths ranged from 2 to 458 m bls, with a median of 101 m bls. Historical data were obtained from five sources: the USGS National Water Information System (U.S. Geological Survey, 2021), the Water Replenishment District of Southern California (variously dated, 2019b), the California State Water Resources Control Board Division of Drinking Water (2018), the California State Water Resources Control Board (2017a, 2017b) GeoTracker, and the California Department of Water Resources (2018).
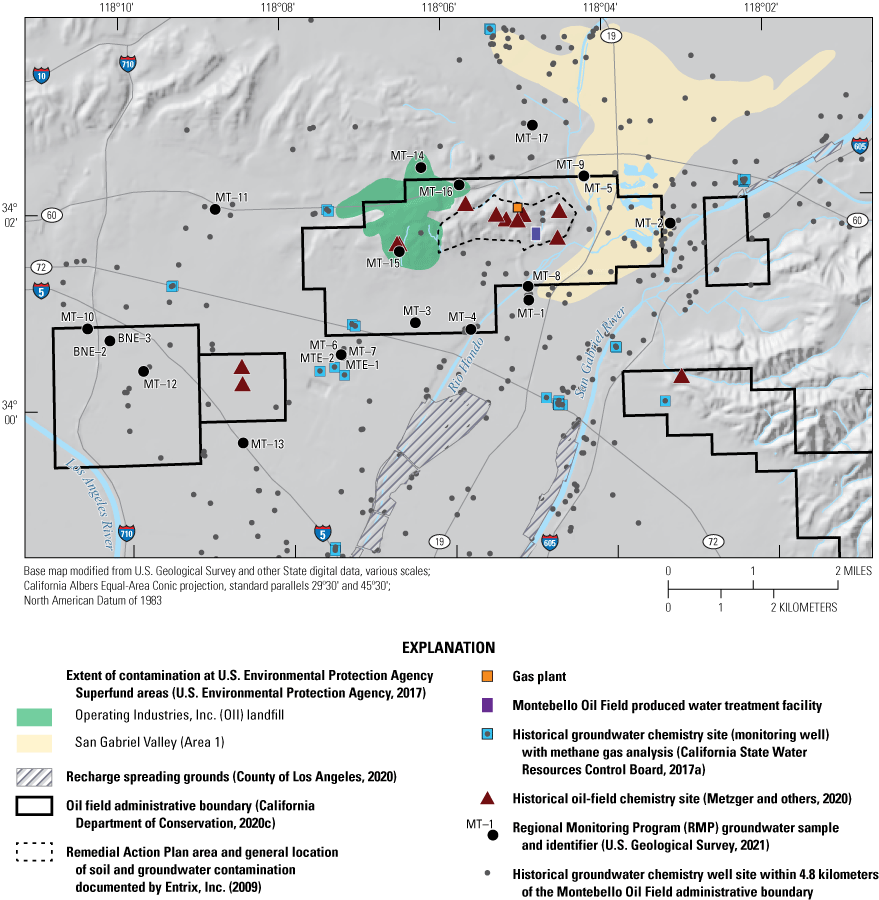
Historical groundwater and oil-field chemistry sample sites in the Montebello Oil Field study area, Los Angeles County, California (California State Water Resources Control Board, 2017a, 2017b; California Department of Water Resources, 2018; California State Water Resources Control Board Division of Drinking Water, 2018; Water Replenishment District of Southern California, variously dated, 2019b; U.S. Geological Survey, 2021).
Historical oil-field water-chemistry data were available from Metzger and others (2020) for 10 oil and gas wells (fig. 4) and 2 injectate samples in the Montebello Oil Field study area. The data were compiled from underground injection control project files of the CalGEM. Depths to the top of sampled oil and gas well perforations ranged from 592 to 2,522 m bls, with a median of 931 m bls. One injectate sample represents produced water obtained from neighboring oil wells and injected into the first zone. The other injectate sample represents blended and treated water obtained from multiple oil and gas wells across the Montebello Oil Field and injected into the eighth zone (fig. 2).
The historical chemistry data were primarily used for mapping the spatial distribution of major ions, selected trace elements, and VOCs as a supplement to the samples collected by the RMP, which had more analytes and lower LDLs but were only available for a limited number of sites. When using historical major ion and trace element data for this study, the most recent sample with complete data was generally used because it was closest in time to the samples collected for this study and substantial changes to those chemical constituents through time were unexpected. For VOCs and gas data, all historical samples at each well were used to evaluate if there had been a detectable concentration in any sample in time. This approach was used to best determine the locations where groundwater has been affected by VOCs and gases regardless of subsequent treatment or attenuation. The LDLs for VOCs obtained from historical data sources were often substantially higher than for RMP samples, typically by about an order of magnitude. To help minimize the effect of variable LDLs from different data sources and laboratory methods, a single benzene, toluene, ethylbenzene, and xylenes (BTEX) compound (benzene) was evaluated using a common concentration level of 0.5 microgram per liter (µg/L; the most commonly used LDL from historical groundwater chemistry data). Benzene was chosen for this assessment because it was the most commonly detected BTEX compound in RMP samples.
Potential Explanatory Factors
Newly collected RMP and historical water-sample data were compared to explanatory factors that have the potential to affect water quality. Data for these factors were compiled from many sources and included measures of oil and gas well density and age; occurrence of oil and gas in sediments above commercial oil- and gas-producing zones; water production and injection volumes (figs. 3C, 3D) and vertical fluid pressure differences; oil and gas well construction and integrity issues; other anthropogenic sources of analyzed constituents; depositional environments of aquifer sediments; and directions of groundwater flow. Aquifer geochemical conditions and groundwater ages determined from historical and RMP samples also were factors for understanding sources and migration pathways of chemical constituents in groundwater.
Records for 160 oil and gas wells from the California Department of Conservation (2018, 2020c) within 500 m of RMP sampled wells were analyzed to assess density, ages, integrity issues (table 2; appendix 3, table 3.1), and height of cement within the well annulus (space between the casing and the formation). A 500-m radius might not represent the distance in which oil and gas wells could affect groundwater quality but provides a consistent approach for evaluating potential relations of oil and gas development to groundwater quality in sampled wells and is a radial distance for assessing relations to surrounding land use that has been used by other groundwater studies (Johnson and Belitz, 2009). The height of cement within the well annulus was estimated based on casing diameter, borehole diameter, casing bottom, and the volume of cement and cement additives used (appendix 4). These data were available for 90 oil and gas wells (fig. 5), representing construction dates between 1922 and 1984. Calculated annular cement heights were compared with operator-reported heights that were available for a subset of the wells to assess the accuracy of the calculated results.
Table 2.
Oil and gas well characteristics within 500 meters of groundwater wells sampled for the Regional Monitoring Program in the Montebello Oil Field study area, Los Angeles County, California, 2014 and 2017–18 (California Department of Conservation, 2020c).[>, greater than; P, plugged; OG, oil and gas production well; —, not applicable; A, active]
Sample identifier (fig. 1) |
Distance to nearest oil and gas well, in meters |
Status of nearest oil and gas well | Type of nearest oil and gas well | Number of abandoned oil and gas wells | Number of active or idle oil and gas wells | Number of injection wells | Number of dry holes | Number of oil and gas wells with recorded well age | Minimum age of oil and gas wells, year drilled | Maximum age of oil and gas wells, year drilled | Mean age of oil and gas wells, year drilled | Number of oil and gas wells drilled before 1976 | Number of oil and gas wells abandoned before 1976 | Number of oil and gas wells with documented well-integrity problem(s)1 | Number of oil and gas wells with documented well integrity problem(s) that potentially intersect groundwater resource zone 1 | Number of mud logs | Fluid volume injected, 1977–2017, in cubic meters |
---|---|---|---|---|---|---|---|---|---|---|---|---|---|---|---|---|---|
MTE-1 | >500 | P | OG | — | — | — | — | — | — | — | — | — | — | — | — | — | — |
MTE-2 | >500 | P | OG | — | — | — | — | — | — | — | — | — | — | — | — | — | — |
BNE-2 | 128 | P | OG | 19 | 0 | 0 | 0 | 19 | 1947 | 1962 | 1956 | 19 | 1 | 1 | 1 | 0 | 0 |
BNE-3 | 128 | P | OG | 19 | 0 | 0 | 0 | 19 | 1947 | 1962 | 1956 | 19 | 1 | 1 | 1 | 0 | 0 |
MT-1 | 97 | P | OG | 4 | 0 | 0 | 0 | 4 | 1920 | 1956 | 1936 | 4 | 4 | 4 | 3 | 1 | 0 |
MT-2 | 220 | P | OG | 1 | 0 | 0 | 0 | 1 | 1935 | 1935 | 1935 | 1 | 1 | 1 | 0 | 0 | 0 |
MT-3 | 266 | P | OG | 12 | 0 | 0 | 0 | 6 | 1939 | 1940 | 1939 | 6 | 6 | 0 | 0 | 0 | 0 |
MT-4 | 376 | P | OG | 3 | 0 | 0 | 0 | 3 | 1919 | 1959 | 1933 | 3 | 3 | 2 | 2 | 0 | 0 |
MT-5 | 411 | P | OG | 5 | 0 | 0 | 0 | 4 | 1919 | 1950 | 1927 | 4 | 2 | 1 | 1 | 0 | 0 |
MT-6 | >500 | P | OG | — | — | — | — | — | — | — | — | — | — | — | — | — | — |
MT-7 | >500 | P | OG | — | — | — | — | — | — | — | — | — | — | — | — | — | — |
MT-8 | 242 | P | OG | 5 | 0 | 0 | 0 | 5 | 1920 | 1952 | 1932 | 5 | 5 | 3 | 3 | 0 | 0 |
MT-9 | 422 | P | OG | 4 | 0 | 0 | 0 | 3 | 1919 | 1950 | 1929 | 3 | 1 | 1 | 1 | 0 | 0 |
MT-10 | 328 | P | OG | 4 | 0 | 0 | 0 | 4 | 1947 | 1957 | 1957 | 4 | 4 | 1 | 1 | 0 | 0 |
MT-11 | >500 | P | OG | — | — | — | — | — | — | — | — | — | — | — | — | — | — |
MT-12 | 66 | P | OG | 9 | 6 | 0 | 0 | 15 | 1947 | 1956 | 1955 | 15 | 1 | 1 | 1 | 0 | 0 |
MT-13 | >500 | P | OG | — | — | — | — | — | — | — | — | — | — | — | — | — | — |
MT-14 | 163 | P | OG | 2 | 0 | 0 | 0 | 2 | 1946 | 1969 | 1958 | 2 | 2 | 1 | 1 | 1 | 0 |
MT-15 | 71 | A | OG | 55 | 39 | 1 | 0 | 93 | 1917 | 2013 | 1948 | 81 | 41 | 20 | 9 | 0 | 217,523 |
MT-16 | 270 | P | OG | 7 | 0 | 0 | 0 | 7 | 1929 | 1948 | 1942 | 7 | 4 | 1 | 1 | 0 | 0 |
MT-17 | 146 | P | OG | 2 | 0 | 0 | 0 | 2 | 1920 | 1922 | 1921 | 2 | 2 | 2 | 2 | 0 | 0 |
See appendix 3 (table 3.1) for details.
Mud logs obtained during the well-drilling process (available from the California Department of Conservation [2020a]) were used to identify the occurrence of oil and gas in drilling mud and cuttings at intervals shallower than (or areas outside) economically productive oil and gas zones (fig. 5). Gas chromatograph instruments determined individual gas components (methane, ethane, propane, butane, and pentane; Crain, 2010) and hydrocarbon fluorescence measured oil as a percentage of mud-log cuttings (Swanson, 1981). Some mud logs also identify traces of hydrocarbons, such as tar, from visual inspection of cuttings. In addition to mud logs, some oil and gas well drilling records were reviewed to determine if petroleum hydrocarbons were present in formations shallower than oil- and gas-producing zones (California Department of Conservation, 2020c).
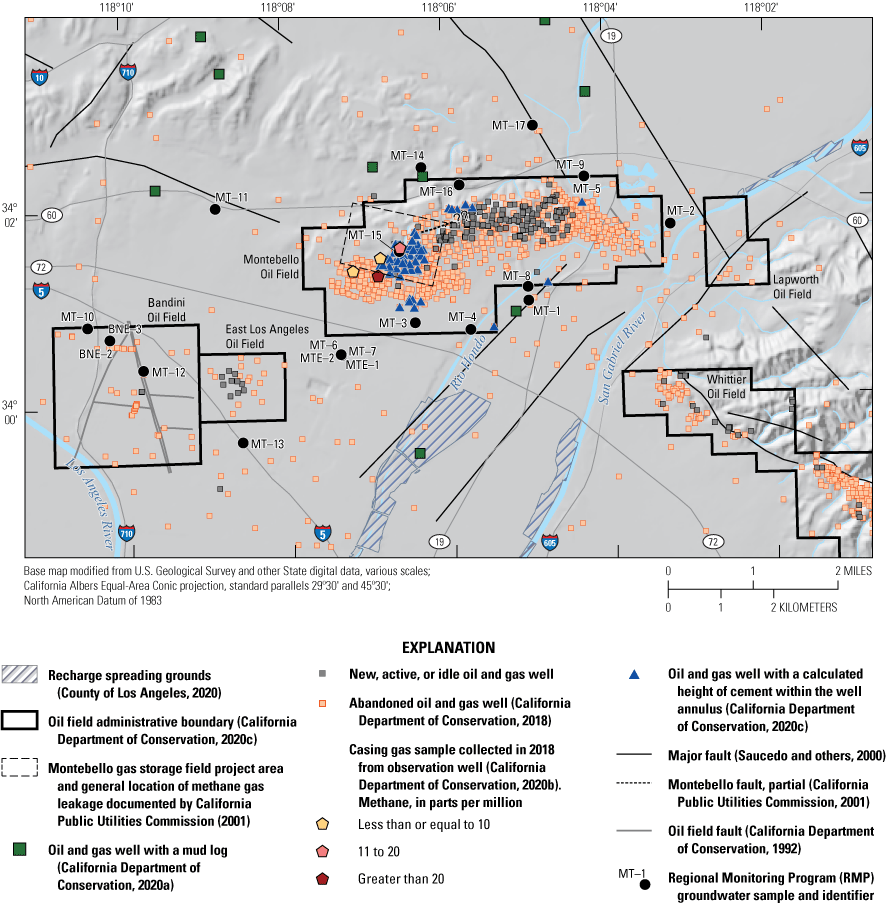
Potential sources of petroleum hydrocarbons associated with subsurface oil field and other activities in the Montebello Oil Field study area, Los Angeles County, California.
Spatial patterns of the fluid volume balance (the difference in volume of produced oil and water and injected water and steam) from January 1977 to February 2018 (California Department of Conservation, 2020c) was assessed for the Montebello Oil Field to estimate areas where positive fluid balances would cause positive pressure and present a risk factor for fluid migration (Shimabukuro and others, 2019). In this analysis, fluid production and injection volumes associated with individual wells were distributed to a small surrounding area near each well; a two-dimensional Gaussian function, to allow summing of volume contributions from individual wells, was used. The spatially summed difference between the injected and produced volumes was then used to create a spatial distribution of fluid volume balances expressed as net fluid heights.
Idle well-fluid levels (appendix 1) that were measured in 82 oil and gas wells across the study area from 1991 to 2017 were available from the California Department of Conservation (2020c). These data were used in three ways: (1) 13 measurements (from 2015 through 2017) were compared with areas of positive and negative fluid volume balances, (2) 14 measurements (in 2015) were compared with water-level elevations in overlying groundwater zones during the same year (Water Replenishment District of Southern California, 2016), and (3) 11 measurements (from 2003 through 2014) within 500 m of sample BNE-3 (appendix 3, table 3.3) were compared with the groundwater level in that sampled well.
Hydrogeologic factors were compiled to examine their potential effect on groundwater quality. Available data about sources of recharge, direction of groundwater flow (fig. 1), distance along flow paths inferred from groundwater-level elevation contours (Reichard and others, 2003; Entrix, Inc., 2009; Ponti and others, 2014; Water Replenishment District of Southern California, 2016), and groundwater residence times were considered when interpreting detections of petroleum hydrocarbons, salinity, and other chemical constituents. A three-dimensional chronostratigraphic model developed by Ponti and others (2014) was used to determine whether the dominant depositional environment of sediments was marine or nonmarine at well-perforation depths (table 1).
Potential sources of anthropogenic contaminants not associated with oil and gas development included two U.S. Environmental Protection Agency (EPA) Superfund areas (see the “Potential Surface Sources and Migration Pathways” section), locations of soil or groundwater State Water Board Cleanup Sites (California State Water Resources Control Board, 2019b), and Toxics Release Inventory sites with a BTEX release in 2018 (U.S. Environmental Protection Agency, 2020a; fig. 6).
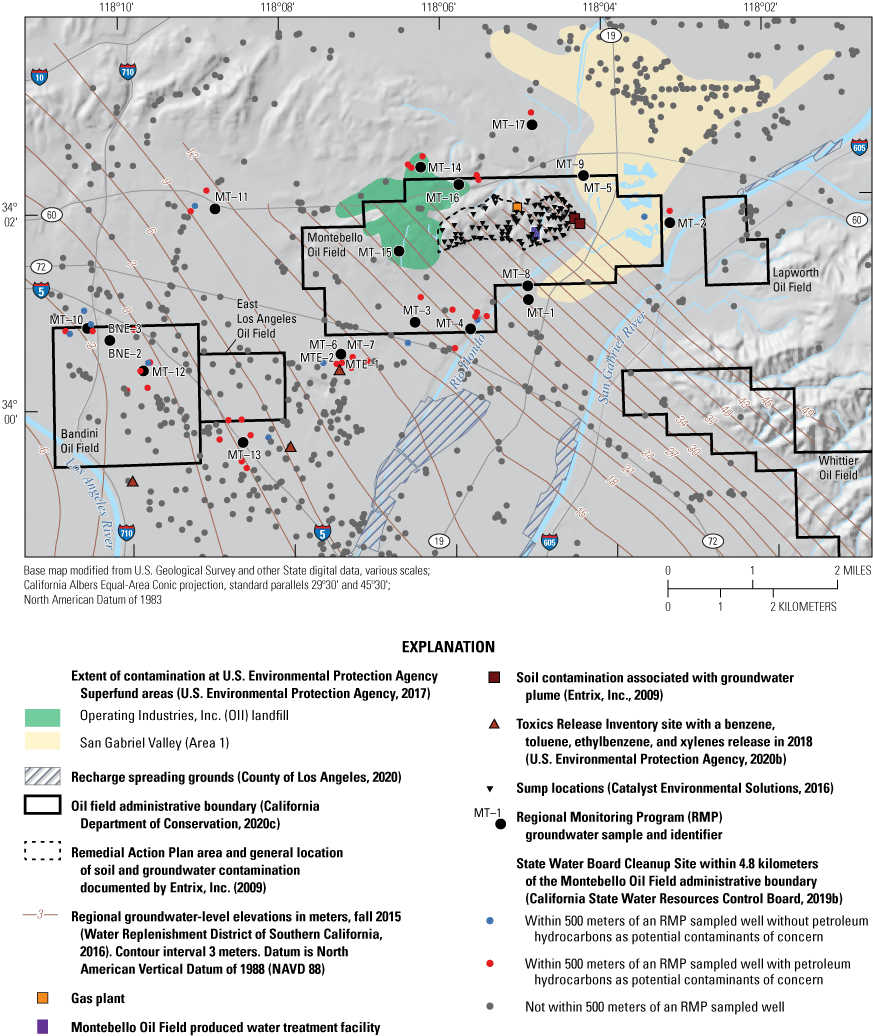
Potential sources of petroleum hydrocarbons associated with oil and gas development and other activities at or near the land surface in the Montebello Oil Field study area, Los Angeles County, California.
Factors That Could Affect Groundwater Quality
This section describes the distribution of factors that could affect groundwater quality. The information provides context for interpreting results of groundwater chemistry data in the “Groundwater Quality near the Montebello Oil Field” and “Potential Sources and Migration Pathways of Chemical Constituents” sections of this report.
Potential Subsurface Sources and Migration Pathways
Injection well pressures, historical depletion of reservoir pressures and any resulting subsidence, and the distribution of net volumes of injection and production in oil reservoirs in the Montebello Oil Field are factors to consider in assessing the potential for fluid migration out of oil- and gas-production zones. Swanson (2018) described conditions in water-flooded oil fields in the Los Angeles Basin, including Montebello, that were operating near injection capacity. Those oil fields have many wells operating near or at the maximum allowable surface pressure, which is approximately 95 percent of the formation fracture pressure. Because injection well efficiencies change through time as wells become clogged (Swanson, 2018), injection rates must be reduced to keep pressures below maximum allowable values until wells are periodically stimulated, using acid washing to remove clogs. Therefore, injection rates and well efficiencies require careful management. Sustained pressures above the formation fracture pressure can increase the risk of fluid migration away from injection sites. Large amounts of injected water also can flush oil-producing formations and change the chemical character of the fluids in the oil reservoir (McMahon and others, 2018; Tyne and others, 2021). However, injected water in the Montebello Oil Field is recycled produced water, so the chemical differences between formation water and injected water are primarily expected to be limited to changes in concentrations of dissolved and noble gases and in oxidation-reduction conditions because of potential atmospheric exposure.
Volumes of injection and reservoir pressures have changed over time in the Montebello Oil Field. Before 1998, produced water was handled through a combination of injection (California Department of Conservation, variously dated), evaporation in disposal ponds, and conveyance to the ocean through pipes (Piper and Garrett, 1953). As a result, injection volumes were smaller than production volumes (fig. 7), potentially causing decreases in reservoir pressure. Sustained decreases in reservoir pressure have resulted in land subsidence in some California oil fields, which can induce well-integrity failures (Myer and others, 1996; Dale and others, 2000). However, based on available Global Positioning System station measurements and calculated estimates, it is likely that any land subsidence in the Montebello Oil Field is no greater than 2 inches (California Public Utilities Commission, 2001; California Department of Water Resources, 2021). The extent of subsidence-related well-integrity issues has not been analyzed in the Montebello Oil Field. Since 1998, the volume of produced water has increased substantially and has mostly been reused for water flooding (fig. 7) to enhance oil production. Part of that produced water had likely been previously injected. Some produced water from the deeper gas storage zone is treated and discharged to the Los Angeles County Sanitation District industrial sewer system or sold to other field operators (California Public Utilities Commission, 2001).
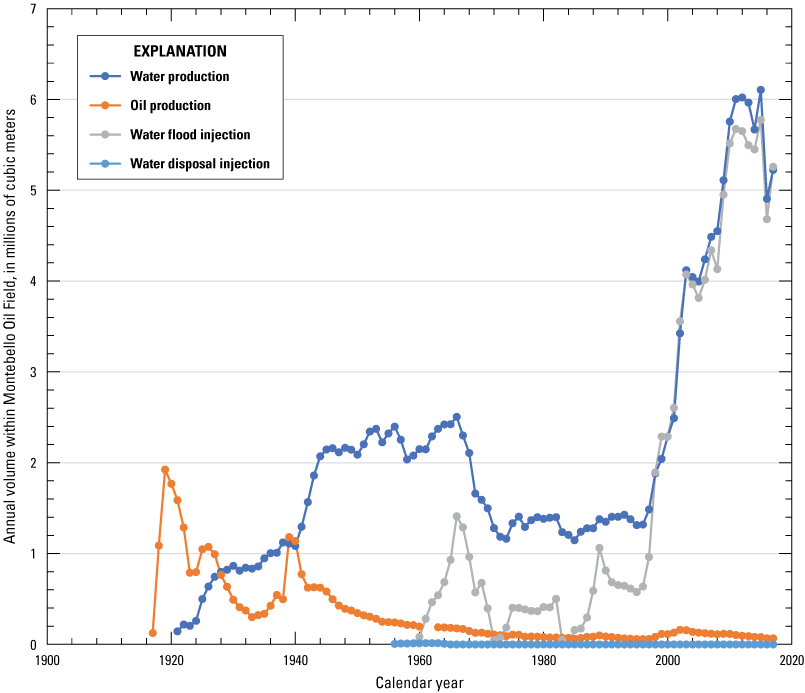
Annual oil production, water production, and water injection volumes, Montebello Oil Field, Los Angeles County, California, 1917–2017 (California Department of Conservation, variously dated).
Production and injection data were available for individual wells from January 1977 to February 2018. Those data, combined with the elevation of the uppermost screened interval for about half of the wells, indicated that produced water was primarily injected into the same horizontal and vertical zone as most of the extracted waters (California Department of Conservation, 2020c; figs. 3C, 3D). About 70 percent of wells with available well-construction data in the Montebello Oil Field study area have an uppermost screened interval shallower than 1,400 m bls; for these wells, the volume of water produced was 48 million m3, and the volume of water injected was 40 million m3. For wells with an uppermost screened interval deeper than 1,400 m bls, the volume of water produced was 5 million m3, and the volume of water injected was 0.2 million m3.
Spatial patterns of the fluid volume balance (the difference in volume of produced oil and water and injected water and steam) for January 1977 to February 2018 (California Department of Conservation, 2020c) were assessed for the Montebello Oil Field to estimate localized areas where fluid imbalances would cause positive pressure and present a risk factor for fluid migration (Shimabukuro and others, 2019). These measurements serve as a proxy for hydraulic heads, which are often difficult to obtain. For most of the field, there is a net negative fluid balance (net fluid height less than zero) where more fluid has been produced than injected (fig. 8). Smaller areas of net positive fluid balance (net fluid height greater than zero) are on the eastern and southern areas. When compared with idle well fluid levels, areas with net positive fluid balances are expected to correspond with higher pressures or shallower fluid levels in idle wells unless a net negative fluid balance existed before 1977. Limited available idle well fluid levels from 2015 through 2017 (California Department of Conservation, 2020c) indicated that idle well fluid levels in the area of positive fluid balance were, on average, shallower (mean elevation below sea level referenced to the North American Vertical Datum of 1988 [NAVD 88] =246 m, n=6) than the idle fluid levels in areas of negative fluid balances in the field (mean elevation below sea level referenced to NAVD 88 =364 m, n=7). This approach does not include effects of activities before 1977, spatially heterogeneous sediments, or effects of dissolved gas but can indicate areas where fluid migration risk could be more likely to exist. This assessment for the Montebello Oil Field lacked complete oil field pool codes and well-perforation data that would provide a more detailed assessment of water production and injection imbalances in individual pools and with depth.
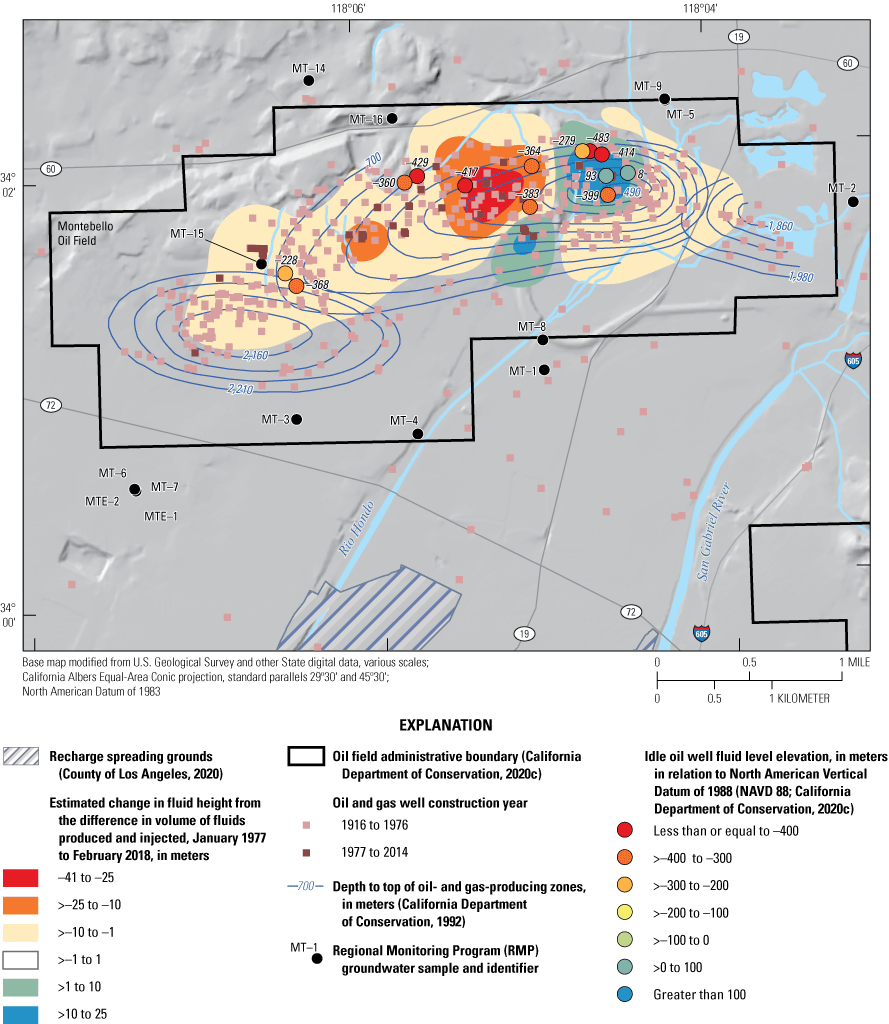
Estimated change in fluid height from the difference in volume of fluids produced and injected (California Department of Conservation, 2020c), Montebello Oil Field, Los Angeles County, California, January 1977 to February 2018.
Higher hydraulic heads in oil- and gas-producing formations, compared to overlying groundwater, indicate the potential for upward migration of water from those formations into groundwater. The idle well-fluid levels near the Montebello Oil Field generally were lower than water-level elevations in overlying groundwater zones, indicating that vertical hydraulic gradients are downward, at least in the modern era, and downward flow would happen if there was a hydraulic connection between the two zones (Water Replenishment District of Southern California, 2016; California Department of Conservation, 2020c). These limited available data indicate that water from oil- and gas-producing formations likely is not moving upward to groundwater during current (2018) conditions. However, literature from the Los Angeles Basin indicates that upward movement of fluids from thermogenic hydrocarbon source rocks to overlying formations could have happened through geologic time (Jung and others, 2015). These processes have produced oil seeps and shallow oil deposits in parts of southern California (Hodgson, 1987; Hornafius and others, 1999); however, the magnitude and occurrence of upward fluxes of fluids from deep formations to sediments overlying oil- and gas-producing formations (through geologic time) in the study area are unknown.
Groundwater used for supply typically is separated from oil- and gas-producing formations by geologic layers that limit fluid movement between the two zones. The median vertical distance between petroleum resource development (top of oil well perforations) and groundwater (bottom of groundwater well perforations) in the Montebello Oil Field is about 650 m (fig. 3A). However, wells and dry holes constructed or abandoned with improper sealing, deteriorated wells, and well-integrity issues may facilitate migration of fluids from oil- and gas-producing formations to shallower zones (Wisen and others, 2020). Movement of fluids between geologic intervals, especially in the upper oil-producing zones, has been considered a possibility in the Montebello Oil Field because of these potential issues (Stolz, 1939; California Public Utilities Commission, 2001). Of the 21 RMP sampled wells, 15 were within 500 m of abandoned oil and gas wells and 2 were near active or idle oil and gas wells (table 2).
A limited well-integrity assessment of oil and gas wells near RMP samples was completed using CalGEM oil and gas well records (California Department of Conservation, 2020c). Well records indicated potential pathways for fluids from oil- and gas-producing zones to groundwater were documented, but data were insufficient to identify any effects on groundwater quality. Well-integrity issues included casing damage or collapse at depth, open holes, and a blowout (appendix 3, table 3.1). Of the 160 oil and gas wells within 500 m of groundwater wells sampled by the RMP, 35 (22 percent) had a well-integrity issue, although not all intersect groundwater resource zones (table 2; appendix 3, table 3.1). Of these oil and gas wells, 20 had a reported integrity issue potentially intersecting groundwater resource zones, defined as being shallower than the base of freshwater approximately estimated by CalGEM (California Department of Conservation, 2020a). Two oil and gas wells had an observed surface expression of a fluid leak. A surface expression is the flow of oil, water, or steam to the land surface at or around the well. Surface expressions indicate an integrity issue in the well, but the potential intersection of these issues with groundwater is unknown. Of the oil and gas wells analyzed, 13 had well-integrity issues reported at depths within oil- and gas-producing zones, below the groundwater resource zone, that may have low risk of affecting groundwater if the wells have an annular cement seal (cement in the annular space between the casing and the formation) between the oil- and gas-producing and groundwater-resource zones.
Well-construction practices have improved through time with respect to protecting groundwater (California Public Utilities Commission, 2001; King and King, 2013), and regulations on the oil and gas industry that specifically targeted protecting groundwater resources were enacted in the 1970s (California Department of Conservation, 2019a). Before regulations were enacted, some oil and gas well operators in the Los Angeles Basin filled wells and dry holes with construction debris and covered them with soil at the land surface. In addition, well casings and cement seals can degrade over time (Dusseault and others, 2000, 2014; California Public Utilities Commission, 2001; King and King, 2013; Ingraffea and others, 2014). Thus, older wells may be more likely to have inadequate protection for migration of fluids from hydrocarbon-bearing formations even when constructed with current (2018) protective measures.
To supplement the limited well-integrity data, about half of the oil and gas well records (452 wells) in the Montebello Oil Field were reviewed to determine well ages (California Department of Conservation, 2020c). Risk of fluid migration may not coincide with a specific well age, but 1976 was used as an approximate year for classifying oil and gas wells with the possibility of higher risk associated with older wells. This approach is supported by the well-integrity assessment results; 33 of the 35 wells with an integrity issue were constructed before 1976 (appendix 3, table 3.1). Of the 452 wells examined for age, 88 percent (400 wells) were constructed before 1976, and of those, 41 percent (165 wells) were abandoned before 1976. Additionally, some wells constructed before 1976 are in areas of the oil field that have a net positive fluid balance (fig. 8), potentially creating risk for oil-field fluid migration if those wells have integrity issues or were improperly constructed or abandoned.
Another approach for assessing potential pathways for upward movement of water from oil-bearing formations or overlying sediments with poor water quality or oil and gas shows is to determine if oil and gas well-casing intervals have gaps in the annular cement at the time of construction. Gaps in the annular cement can allow any escaped fluids from a well-integrity failure to easily move along the annular space to other geological intervals (Dusseault and others, 2000; Watson and Bachu, 2009; Davies and others, 2014). The limited analysis for oil-field wells within 500 m of RMP samples indicates gaps in calculated annular cement between oil- and gas-producing zones and fresh groundwater (typically less than 600 m bls; appendix 3, table 3.1) for many wells, regardless of the well-construction date (fig. 9); however, deeper oil and gas wells completed after 1964 generally have longer cement intervals than older wells. The calculated annular cement heights were similar to the operator-reported values for most of the oil and gas wells with an operator-reported cement interval available for comparison. The oil and gas wells shown in figure 9 were not distributed evenly across the oil field because RMP sample MT-15 was the only sample collected in an area near many oil and gas wells (fig. 5). Thus, these results primarily represent well-construction practices in an area west of most production and injection activities. However, if those practices are consistent with other wells in the Montebello Oil Field, then oil and gas well boreholes represent a potential pathway for fluids to migrate away from hydrocarbon-bearing formations if a well-integrity issue exists.
A limitation of using gaps in annular cement of oil and gas well-casing intervals to assess potential upward movement of water is that the annular cement record represents a construction record and conditions that existed before the well was abandoned. A well with an uncemented annulus may later be filled during abandonment but would not be accounted for in this assessment. The oil- and gas-well borehole conditions before abandonment could have been present for several decades between well installation and abandonment.
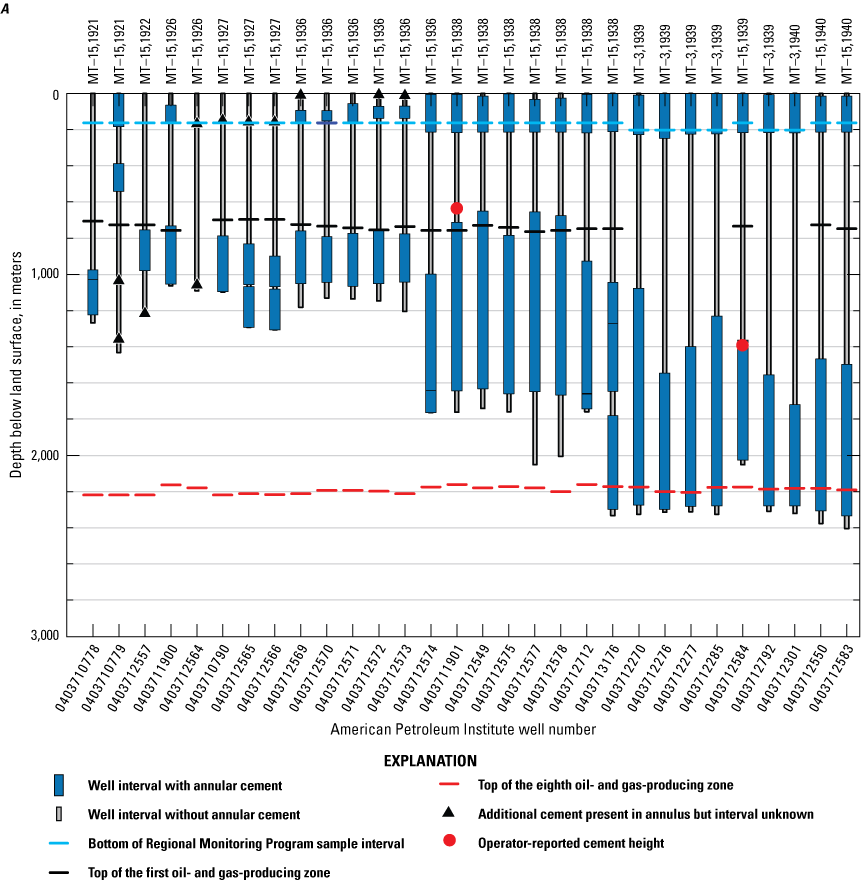
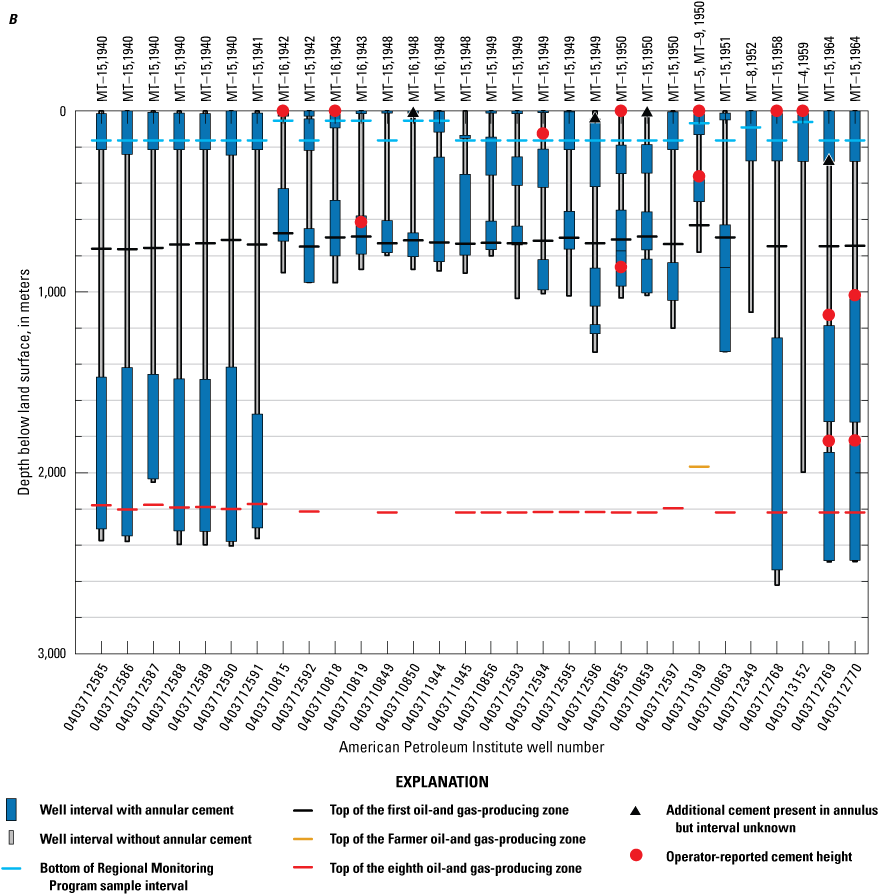
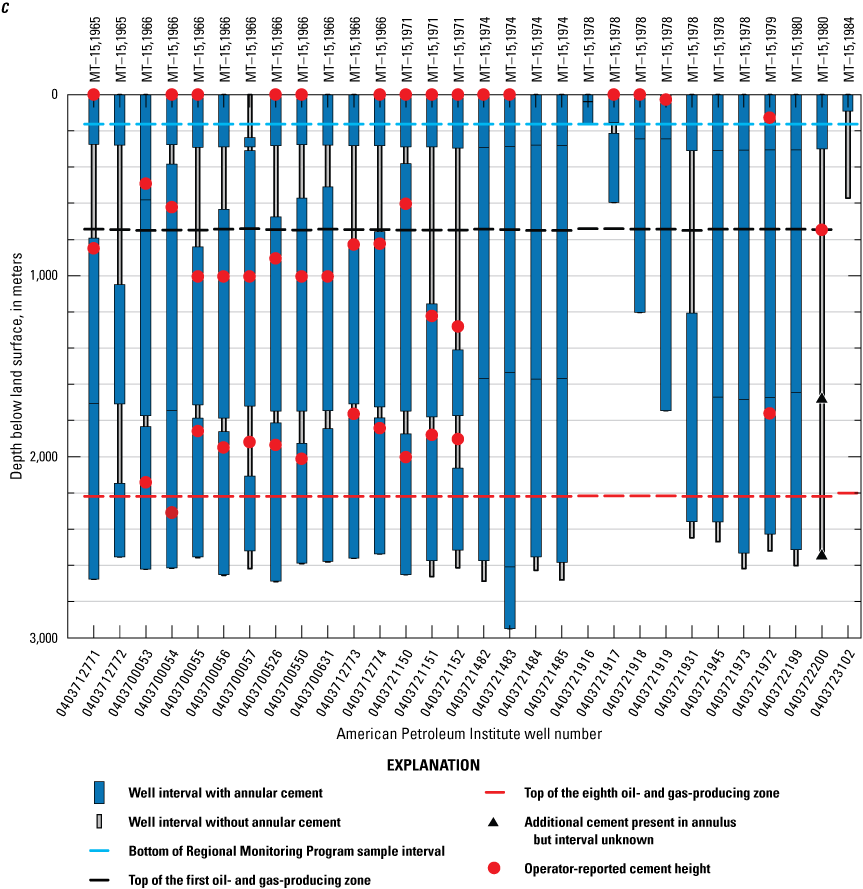
Calculated annular cement intervals for Montebello Oil Field oil, gas, and observation wells within 500 meters of a Regional Monitoring Program sample, Los Angeles County, California. Overlapping well casings of different diameters are not depicted. Top of oil- and gas-producing zones at well locations were estimated by overlying well locations with contours of the top of oil- and gas-producing zones published by the California Department of Conservation (1992).
Natural migration of fluids from deep hydrocarbon-bearing formations to overlying groundwater could result from preferential pathways, such as fault planes, fracture systems, or geologic unconformities that intersect porous and permeable formations or from diffuse upward flow through zones where low permeability layers that cap hydrocarbon-bearing formations are absent or thin. Natural upward fluxes from hydrocarbon-bearing formations are typically considered to be limited because overburden pressure minimizes the effects of fractures and faults (California Public Utilities Commission, 2001). However, information regarding upward fluxes of fluids due to natural migration over geologic time in the Montebello Oil Field is unknown. Shallower Pleistocene and Holocene deposits are more permeable than deeper formations (California Public Utilities Commission, 2001) and could allow migration of any oil and gas present in those zones due to geologic oil or gas shows in these sediments or upward migration from deeper formations. The Montebello fault (fig. 5) and other potentially active faults (locations unknown) are in the Montebello Oil Field but are not areally extensive (California Public Utilities Commission, 2001); fluxes of fluids from hydrocarbon-bearing formations along faults are unknown.
The natural occurrence of petroleum hydrocarbons and other chemical constituents outside of oil- and gas-producing zones is common in California (Hodgson, 1987) and has potential to affect groundwater resources. In some oil fields in California, mud log data show evidence of naturally occurring oil and gas shows in sediments overlying oil- and gas-producing zones (Rosecrans and others, 2021; Anders and others, 2022) that indicate upward migration of oil and gas into shallow sediments through geologic time. These shallow oil and gas shows could contribute to the presence of some chemical constituents in groundwater. Mud logs were available from nine oil and gas wells in the study area (fig. 5; appendix 3, table 3.2). All nine mud logs were outside of oil field administrative boundaries; two mud logs were within 500 m of RMP sampled wells (MT-1 and MT-14). Mud log data and evidence of oil and gas shows in sediments overlying the Montebello Oil Field are limited but indicated that traces of tar and oil shows were detected in sediments in the study area at depths greater than or equal to 457 m bls (California Department of Conservation, 2019b). In addition, California Department of Conservation (2007) records indicate the presence of hydrocarbons in the aquifer at the base of freshwater, historically interpreted to have a total dissolved solids (TDS) concentration of less than approximately 3,000 mg/L. Another potential source of elevated concentrations of inorganic chemical constituents is the presence of paleo-seawater in marine sediments that have not been flushed with meteoric water (Piper and Garrett, 1953).
Gas leaks have been documented at several locations in the western part of the Montebello Oil Field (fig. 5; California Public Utilities Commission, 2001), but the total extent and prevalence are unknown. The California Public Utilities Commission (2001) noted that operating pressure in the gas storage zone (as of 2001) was about 40-percent lower than initial reservoir pressure in 1937, indicating that fluids should not migrate out of the gas storage zone. Yet, gas leaks were detected in residential areas between the 1970s and 1990s (fig. 5; California Public Utilities Commission, 2001). During a routine leakage survey in the 1970s, stored thermogenic gas (identified from the presence of helium) was discovered in soils of a residential neighborhood. The California Public Utilities Commission proposed two abandoned oil and gas wells and shallow fault zones as possible migration pathways for the gas. The soil gas was successfully treated to undetectable levels using extraction wells in the 1980s. In 1983, a well survey reported that several abandoned wells were leaking stored thermogenic gas (California Public Utilities Commission, 2001). The Southern California Gas Company suspected the leaks were caused by a combination of pressure differentials between deep and shallower zones and compromised cement seals of abandoned oil and gas wells. The abandoned wells were resealed with cement and procedures were improved to prevent additional leakage of stored gas. Leaking gas was discovered in two more abandoned wells; one leak was detected from 1984 to 1987 and another leak was detected in 1999 (California Public Utilities Commission, 2001). Gas probes detected unprocessed (not stored) thermogenic gas, with no helium or high concentrations of heavy hydrocarbons, along the border of the nearby Operating Industries, Inc. (OII) landfill in 1999 (fig. 6). Nearby features were described as wells located under the landfill (fig. 6) and the Montebello fault (fig. 5) to the east of the landfill (California Public Utilities Commission, 2001). In 2018, gas was detected in a shallow oil and gas observation well (less than 200 m bls) and triggered more frequent monitoring (California Department of Conservation, 2020b). The gas contained propane and heavier gases but low concentrations of helium, indicating unprocessed thermogenic gas. The cause of elevated gas levels in the observation well is currently being examined.
Potential Surface Sources and Migration Pathways
Surface spills or releases of hydrocarbons associated with oil and gas development and other activities at or near the land surface are common near the Montebello Oil Field. These spills can affect groundwater quality through infiltration. Historical unlined produced water disposal ponds and drilling fluid disposal sumps could have affected groundwater quality via infiltration of these fluids to unconfined aquifers. However, locations of drilling fluid disposal sumps in the Montebello Oil Field study area were only available for the Remedial Action Plan area (fig. 6). There were at least 99 sump locations distributed throughout that area, and they have not been used since 2008 (Entrix, Inc., 2009; Catalyst Environmental Solutions, 2016). It is unknown whether those sumps are lined. Additional oil field infrastructure features at the Montebello Oil Field include storage tanks, chemical storage areas, a produced water-treatment facility, and a gas separation and compression plant. Each of these features could be a potential source of petroleum hydrocarbons to soils and groundwater in the event of a fluid spill. Other potential anthropogenic sources of contaminants not associated with oil and gas development include EPA Superfund areas, locations of soil or groundwater State Water Board Cleanup Sites (California State Water Resources Control Board, 2019b), and Toxics Release Inventory sites with a BTEX release (fig. 6; U.S. Environmental Protection Agency, 2020a).
Two EPA Superfund areas are in the Montebello Oil Field: (1) the San Gabriel Valley and (2) the OII landfill (fig. 6). The San Gabriel Valley Superfund area is on the east side of the Montebello Oil Field. Previous research identified VOCs present at the site, including tetrachloroethylene (PCE), trichloroethylene (TCE), other solvents, petroleum hydrocarbons, and the trihalomethane chloroform (URS Corporation, 2015). A pump-and-treat system was completed on site in 2002 to remediate contaminated groundwater. As of 2011, treatment had minimized migration of contaminants off-site and to supply wells. Although concentrations of PCE were frequently greater than the drinking-water benchmark (U.S. Environmental Protection Agency, 2011), they were consistently declining. The only other contaminants of concern that were regularly detected in 2011 were the solvents TCE and 1,4-dioxane, and concentrations of those compounds generally were below their respective drinking-water benchmarks (U.S. Environmental Protection Agency, 2011). Regional groundwater flows from this superfund area toward the Montebello Oil Field and the Central Basin (fig. 1).
The OII landfill is on the northwest side of the Montebello Oil Field (U.S. Environmental Protection Agency, 2015). Operations as a municipal landfill for the City of Monterey Park (fig. 1), California, began in 1948, accepting residential and commercial refuse, industrial wastes, liquid wastes, and various hazardous wastes. Landfill leachate was detected off-site in 1982, all landfill operations ceased in 1984, and various measures were put in place to control and monitor leachate and landfill gas migration. In 1996, however, the EPA did a risk assessment and concluded that potential human health risks still existed. The constituents of concern included VOCs and semi-volatile organic compounds, pesticides, hydrocarbons, and inorganic constituents in ambient air, groundwater, and off-site soils. These contaminants included methane (microbial) and constituents in common with hydrocarbon-bearing formations, such as BTEX compounds and inorganic compounds (ammonia and barium). As of 2020, concentrations of contaminants of concern generally are decreasing, but 1,4-dioxane, TCE, and cis-1,2-dichloroethene may not reach cleanup goals by the time frame specified in the Record of Decision (U.S. Environmental Protection Agency, 2020b).
During several site assessments in the mid-1990s, soil contamination from petroleum products was discovered near inactive Montebello Oil Field infrastructure (fig. 6; Entrix, Inc., 2009). Soils were remediated where required to achieve acceptable concentration levels determined by the California Regional Water Quality Control Board (Los Angeles Region) and California Department of Fish and Game. A Phase II assessment was completed in 2006 for the areas not included in the scope of the 1990s remediation (Entrix, Inc., 2009). Shallow soil effects from petroleum products were discovered during these assessments, with an estimated soil volume of about 32,000 m3 requiring remediation near well sites, the produced water treatment facility, the gas plant, and other oil-field infrastructure (fig. 6). Deeper, unsaturated sediments containing petroleum products were assumed to be naturally occurring because they were not near infrastructure and, consequently, remediation was not planned. For soil samples collected as part of the Phase II assessment, sample concentrations were compared with California Human Health Screening Levels (Entrix, Inc., 2009, appendix D) and site or regional background concentrations (Chernoff and others, 2008; Entrix, Inc., 2009) to determine possible health risks. Based on this evaluation, total petroleum hydrocarbons, arsenic, and cadmium were considered chemical constituents of concern.
Published information includes several sites with contaminated groundwater in the Montebello Oil Field (Entrix, Inc., 2009). At one location, a plume of contaminated groundwater extending for about 61 m and containing up to 330 mg/L of total petroleum hydrocarbons was associated with soil contamination (fig. 6) discovered during abandonment of two oil and gas wells on the east side of the Montebello Oil Field. The direction of the plume was not described by Entrix, Inc. (2009), but nearby, local groundwater flow is to the southeast in the direction of the Rio Hondo (fig. 1). The affected groundwater was discovered in 1991, and the petroleum hydrocarbons had naturally attenuated by 1997. In another case, contamination near a bioremediation cell necessitated installation of two monitoring wells. Quarterly groundwater sampling of those wells began in 2001; only one recent sample (from May 2008) contained petroleum products (total petroleum hydrocarbon concentration of 0.56 mg/L) as of December 2009 (Entrix, Inc., 2009).
Potential anthropogenic contaminants have been identified at soil or groundwater State Water Board Cleanup Sites (fig. 6; California State Water Resources Control Board, 2019b). Of the 45 State Water Board Cleanup Sites that were within 500 m of an RMP sampled well, 38 were leaking underground storage tank cases and 35 specifically identified petroleum hydrocarbons as potential contaminants of concern. All but two of the RMP sampled wells (MT-1 and MT-8) were within 500 m of at least one of these potential contaminant sources.
Groundwater Quality near the Montebello Oil Field
Groundwater near the Montebello Oil Field was analyzed for VOCs, gas composition, age-dating tracers, and inorganic tracers to determine concentrations of constituents that may be associated with hydrocarbon-bearing formations, among other sources. This section describes the results of RMP and historical sample data to assess the general distribution of groundwater quality across the study area.
Volatile Organic Compounds
Analyzed VOCs were categorized as petroleum hydrocarbon or manufactured compounds (table 3; appendix 3, tables 3.4, 3.5). Petroleum hydrocarbons were detected in 29 percent (6 of 21) of RMP groundwater samples (fig. 10) at concentrations substantially below drinking-water benchmarks (fig. 11; appendix 3, tables 3.4, 3.5; U.S. Environmental Protection Agency, 2018; California State Water Resources Control Board, 2019c; California State Water Resources Control Board Division of Drinking Water, 2020). The most prevalent petroleum hydrocarbons detected were benzene (five samples), 1,2,4-trimethylbenzene (two samples), ethylbenzene (two samples), m-Xylene plus p-Xylene (two samples), o-Xylene (two samples), and 4-isopropyltoluene (one sample; fig. 11; appendix 3, table 3.4). Carbon disulfide (not shown), a naturally occurring or manufactured VOC (Agency for Toxic Substances and Disease Registry, 1996), was detected in four samples, three of which contained hydrocarbons (appendix 3, table 3.5). None of the petroleum hydrocarbon concentrations exceeded 0.1 µg/L, and only 5 of the 14 detected concentrations were equal to or larger than the LDL(s) for the respective compound (fig. 11; appendix 3, table 3.4).
Table 3.
Volatile organic compound and age-dating results for Regional Monitoring Program groundwater samples in the Montebello Oil Field study area, Los Angeles County, California, 2014 and 2017–18 (U.S. Geological Survey, 2021).[ND, not detected above laboratory detection level]
Sample identifier (fig. 1) |
Tritium concentration, in tritium units |
Carbon-14 in dissolved inorganic carbon, in percent modern carbon |
Groundwater age category based on tritium and carbon-14 results (fig. 14) |
Number of manufactured volatile organic compounds detected1 |
Number of petroleum hydrocarbons detected1 |
---|---|---|---|---|---|
MTE-1 | ND | 0.13 | Pre-modern | 0 | 0 |
MTE-2 | 8.84 | 133.56 | Modern | 2 | 0 |
BNE-2 | 8.12 | 102.17 | Modern | 5 | 0 |
BNE-3 | ND | 2.64 | Pre-modern | 0 | 1 |
MT-1 | 5.83 | 94.43 | Modern | 2 | 0 |
MT-2 | 5.74 | 95.95 | Modern | 6 | 0 |
MT-3 | 2.76 | 92.57 | Modern | 3 | 0 |
MT-4 | 6.55 | 96.40 | Modern | 2 | 0 |
MT-5 | 8.21 | 92.76 | Modern | 2 | 1 |
MT-6 | 0.19 | 0.58 | Pre-modern | 0 | 0 |
MT-7 | 4.45 | 93.44 | Modern | 3 | 0 |
MT-8 | 5.14 | 90.53 | Modern | 0 | 0 |
MT-9 | 0.25 | 24.26 | Mixed | 1 | 1 |
MT-10 | 3.67 | 86.94 | Mixed | 8 | 0 |
MT-11 | 1.50 | 73.49 | Mixed | 2 | 0 |
MT-12 | 3.48 | 109.37 | Modern | 10 | 0 |
MT-13 | 4.04 | 102.12 | Modern | 3 | 0 |
MT-14 | ND | 38.12 | Pre-modern | 0 | 0 |
MT-15 | ND | 38.94 | Pre-modern | 0 | 2 |
MT-16 | ND | 107.88 | Mixed | 12 | 5 |
MT-17 | 0.94 | 88.54 | Mixed | 2 | 4 |
Concentrations provided in appendix 3 (table 3.5).
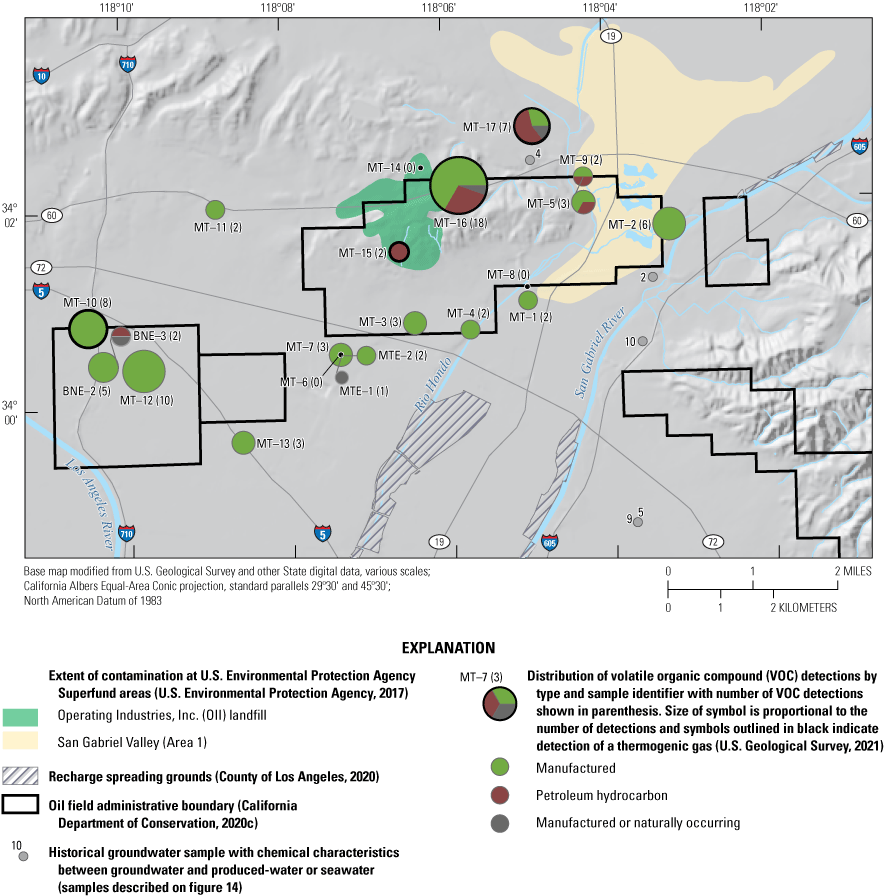
Distribution of volatile organic compounds by type for Regional Monitoring Program groundwater samples in the Montebello Oil Field study area, Los Angeles County, California, 2014 and 2017–18.
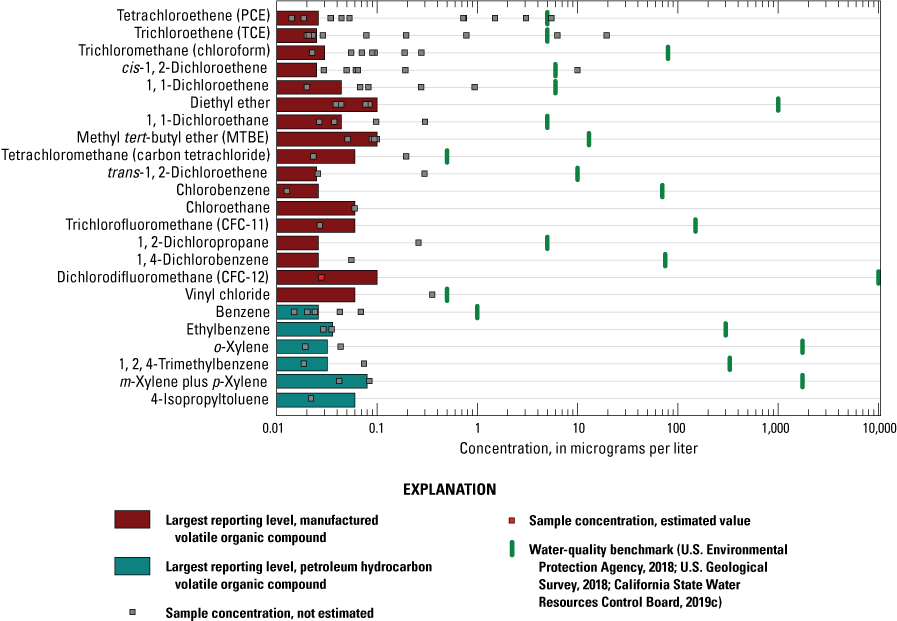
Concentrations of detected volatile organic compounds for Regional Monitoring Program groundwater samples in the Montebello Oil Field study area, Los Angeles County, California, 2014 and 2017–18.
Detected petroleum hydrocarbons in deeper groundwater intervals may be at least partially explained by the presence of naturally occurring hydrocarbons in sediments shallower than commercial oil-producing formations. The limited available mud logs in the study area have evidence of oil shows in sediments at depths as shallow as 357 m bls (appendix 3, table 3.2) in a part of the Bandini Oil Field, near BNE-3, where benzene was detected at a depth of 405–424 m bls (appendix 3, table 3.5; see the “Potential Sources and Pathways of Chemical Constituents” section).
Benzene, toluene, ethylbenzene, and xylenes compounds commonly are measured in groundwater because of their adverse human-health effects (Lawrence, 2006). They also are the most frequently detected VOCs derived from petroleum products (including oil and gasoline) because they have relatively higher solubilities than other hydrocarbons (Lawrence, 2006). For these reasons, BTEX compounds are useful for summarizing the distribution of petroleum hydrocarbon detections from multiple sources. Laboratory detection levels (LDLs) of BTEX compounds for historical data sources were substantially higher than for RMP samples, typically by about an order of magnitude. To help minimize the effect of variable LDLs from different data sources and laboratory methods, a single BTEX compound (benzene, the most commonly detected BTEX compound in RMP samples) was evaluated using a common concentration level of 0.5 µg/L (the most commonly used LDL from historical groundwater chemistry data).
Benzene was detected at concentrations greater than 0.5 µg/L in 18 percent (103 of 585) of wells (historical and RMP data) analyzed for benzene in the Montebello Oil Field study area (California State Water Resources Control Board 2017a, 2017b; California State Water Resources Control Board Division of Drinking Water, 2018; Water Replenishment District of Southern California, variously dated, 2019b; U.S. Geological Survey, 2021; Rodriguez and others, 2022). Detections of benzene greater than the common concentration level (0.5 µg/L) were more frequent at shallower depths (fig. 12); benzene was detected in 12 percent of the 197 wells completed in shallow zones (less than or equal to 122 m bls). Benzene was not detected in any of the 111 wells completed in intermediate zones (between 122 and 305 m bls) nor in any of the 5 wells completed in deep zones (greater than 305 m bls). Of the 272 wells without available depths, 79 had a benzene concentration greater than 0.5 µg/L. Benzene was detected at multiple locations in shallow groundwater, in no apparent spatial pattern, indicating the presence of many sources of benzene at or near the land surface in the study area.
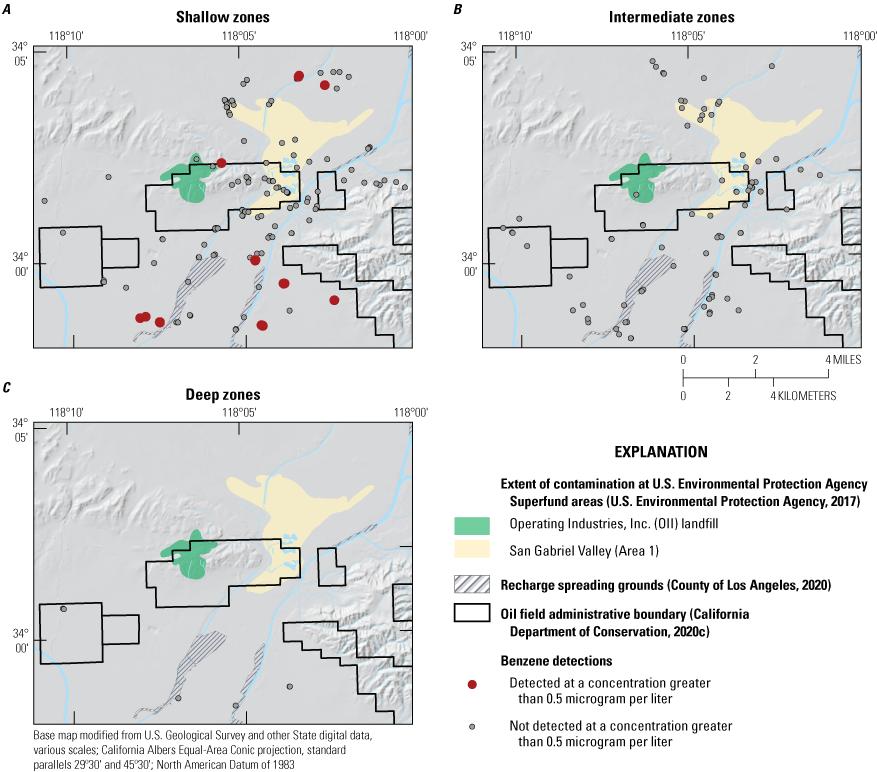
Distribution of benzene detections greater than 0.5 microgram per liter for Regional Monitoring Program groundwater samples in the Montebello Oil Field study area, Los Angeles County, California, 2014 and 2017–18 and historical groundwater samples for A, shallow zones (depths less than or equal to 122 meters below land surface); B, intermediate zones (depths between 122 and 305 meters below land surface); and C, deep zones (depths greater than 305 meters below land surface) (U.S. Geological Survey, 2021; Water Replenishment District of Southern California, variously dated, 2019b; California State Water Resources Control Board Division of Drinking Water, 2018; California State Water Resources Control Board 2017a26, 2017b).
Activities other than oil and gas development have had a larger effect on groundwater quality in the study area with respect to VOCs, even assuming all the petroleum hydrocarbon VOC detections are associated with oil and gas development. Manufactured VOCs were detected in RMP samples more often (15 of 21 RMP samples; fig. 10; table 3) and at larger concentrations (0.0127 to 19.6 µg/L) than petroleum hydrocarbons (appendix 3, tables 3.4, 3.5). Tetrachloroethene (PCE), trichloroethene (TCE), and cis-1,2-dichloroethene were all detected at concentrations larger than water-quality benchmarks for drinking water in samples from one monitoring well (U.S. Environmental Protection Agency, 2018; California State Water Resources Control Board, 2019c); TCE alone was detected at a concentration above the water-quality benchmark in one supply well. This aforementioned supply well is already monitored for VOCs as part of regulatory monitoring, and raw water from this well is treated and blended before distribution as potable water. The concentrations of most manufactured VOCs in RMP samples were well below water-quality benchmarks and many were smaller than LDLs. Most of the detected manufactured VOCs were solvents (42 of the 63 manufactured VOC detections were solvents; appendix 3, table 3.4); other categories of manufactured VOCs detected in RMP samples were trihalomethanes, organic synthesis compounds, gasoline oxygenates, fumigants, and refrigerants. The compounds detected most often were PCE, TCE, and trichloromethane (chloroform). The presence of petroleum hydrocarbons in samples that also contain gasoline oxygenates, such as methyl tert-butyl ether (a common additive to gasoline), could indicate that the source of petroleum hydrocarbons in those samples may be from leaking underground storage tanks and not related to oil and gas production or shallow petroleum hydrocarbons outside of producing zones. Of the 21 RMP samples, 16 were within 500 m of at least 1 State Water Board Cleanup Site (fig. 6) that was a leaking underground storage tank, and almost all those Cleanup Sites had petroleum hydrocarbons included as a contaminant of concern (California State Water Resources Control Board, 2019b). However, petroleum hydrocarbons were not present in the four samples that contained gasoline oxygenates (methyl tert-butyl ether; appendix 3, table 3.5).
The frequent detection of manufactured VOCs is likely related to the long-term urban development of the area. Previous studies have identified relations between the occurrence of manufactured VOCs and urban land use (for example, Zogorski and others, 2006). Studies of VOCs in Los Angeles Basin groundwater have identified the Montebello Forebay (fig. 1), where shallow unconfined groundwater can be directly influenced by overlying land-surface activities, to be a regional recharge area for groundwater and a link to manufactured VOCs downgradient in confined aquifers (Shelton and others, 2001; Dawson and others, 2003; Goldrath and others, 2012). Similarly, manufactured VOCs were widely detected in groundwater underlying the urban area of Bakersfield, California (not shown), in the RMP study of the Fruitvale Oil Field (Wright and others, 2019). Manufactured VOCs have not been detected as frequently in RMP samples collected near oil fields in rural areas (Davis and others, 2018b; Rosecrans and others, 2021; Anders and others, 2022).
Gases
Methane is produced either as a byproduct of the biologic decomposition of organic matter (microbial methane) or in deep geologic formations with high pressure and temperature that produce coal, oil, or natural gas deposits (thermogenic methane; appendix 1; Kulongoski and others, 2018). Microbial methane is produced in highly reducing settings, such as swamps, landfills, or aquifers with geochemically reducing conditions (Whiticar and others, 1986). Thermogenic methane sometimes co-occurs with the heavier gases ethane, propane, isobutane (2-methylpropane), n-butane, isopentane (2-methylbutane), n-pentane, and hexane. The presence of propane and heavier gases is diagnostic of thermogenic contributions to formation gases (Schoell, 1980). Because ethane can also result from microbial processes in some cases, the presence of it is not necessarily diagnostic of thermogenic sources (Taylor and others, 2000).
Dissolved methane was detected in 18 RMP samples, at concentrations ranging from 0.0009 to 110 mg/L and with a median of 0.0085 mg/L (appendix 3, table 3.6). The three samples with a methane concentration greater than 5 mg/L (MT-6, MTE-1, and BNE-3; appendix 3, table 3.6) were from the deepest wells sampled (216, 293, and 424 m bls, respectively; table 1).
Heavier gases in the propane through pentane (C3–C5) range were detected in four of the samples (MT-10, MT-15, MT-16, and MT-17; appendix 3, table 3.6) that contained methane, indicating at least a small fraction of the gases at these sites are derived from thermogenic sources. Concentrations of these gases were small compared to the total volume of dissolved gas (0.0034 mole percent or less). Sample MT-10 was collected from a well to the west of the Montebello Oil Field, in the Bandini Oil Field (fig. 1). Sample MT-10 contained chemical characteristics consistent with a mixture of modern and pre-modern age water (see the “Age-Dating Tracers” section; table 3), manufactured VOCs, and no petroleum hydrocarbons. Sample MT-15 was collected from a deeper well (163 m deep) near oil-field infrastructure (table 2) and contained pre-modern water and petroleum hydrocarbon VOCs, but this sample did not contain manufactured VOCs (appendix 3, table 3.5). Four heavier gases were detected in sample MT-16 and three heavier gases were detected in sample MT-17 (appendix 3, table 3.6). Both samples (MT-16 and MT-17) were collected from relatively shallow wells (less than 60 m deep; table 1) and had a mixture of modern and pre-modern age water (table 3). Sample MT-16 was collected from a well on the north edge of the Montebello Oil Field near the OII landfill (fig. 6). The OII landfill is a possible source of methane and many other constituents, and sample MT-16 included the largest number of manufactured and petroleum hydrocarbon VOC detections of any sample (12 and 5 detections, respectively; table 3; appendix 3, table 3.5). Sample MT-17 was collected from a well near a golf course north of the oil field (fig. 6) and contained manufactured and petroleum hydrocarbon VOCs (table 3; appendix 3, table 3.5). Potential sources of thermogenic gases in samples are discussed in the “Potential Sources and Migration Pathways of Chemical Constituents” section.
The source of methane also can be determined based on the relative abundances of gases and the stable isotopes of hydrogen and carbon in methane (δ2H-CH4, δ13C-CH4; figs. 13A, 13B; appendix 3, table 3.6; Whiticar and others, 1986; McMahon and others, 2017; Kulongoski and others, 2018). Five samples had a methane concentration large enough (at least 0.13 mg/L) to analyze for isotopes, and isotope results indicated that the methane largely originated from microbial sources, most likely through carbon dioxide reduction processes for samples MT-6, MTE-1, BNE-3, and MTE-2 and fermentation processes for sample MT-17 (fig. 13B), and not from thermogenic sources associated with hydrocarbon-bearing formations in the Los Angeles Basin (Jeffrey and others, 1991). These results are mostly consistent with those from reconnaissance sampling of monitoring wells near oil fields that reported high concentrations of methane in some Los Angeles Basin groundwater are primarily derived from natural microbial processes in aquifers rather than thermogenic sources (Kulongoski and others, 2018). However, unlike results from reconnaissance sampling, MT-17 appears to be from fermentation processes. In addition, background methane was present throughout the intervals recorded by mud logs (fig. 5; appendix 3, table 3.2), providing evidence that its presence is common outside of oil- and gas-production zones. Although this approach indicated that methane in samples MT-6, MTE-1, BNE-3, MTE-2, and MT-17 largely originated from microbial sources, the presence of heavier gases in sample MT-17 is consistent with thermogenic sources. These results demonstrate a mixture of multiple sources of constituents in that sample. However, the relative abundances of gases and δ13C-CH4 data in MT-17 show a strong microbial signal, so the thermogenic component appears to be small. In addition, landfill gas and some other shallow gas sources are associated with fermentation (Coleman and others, 1995).
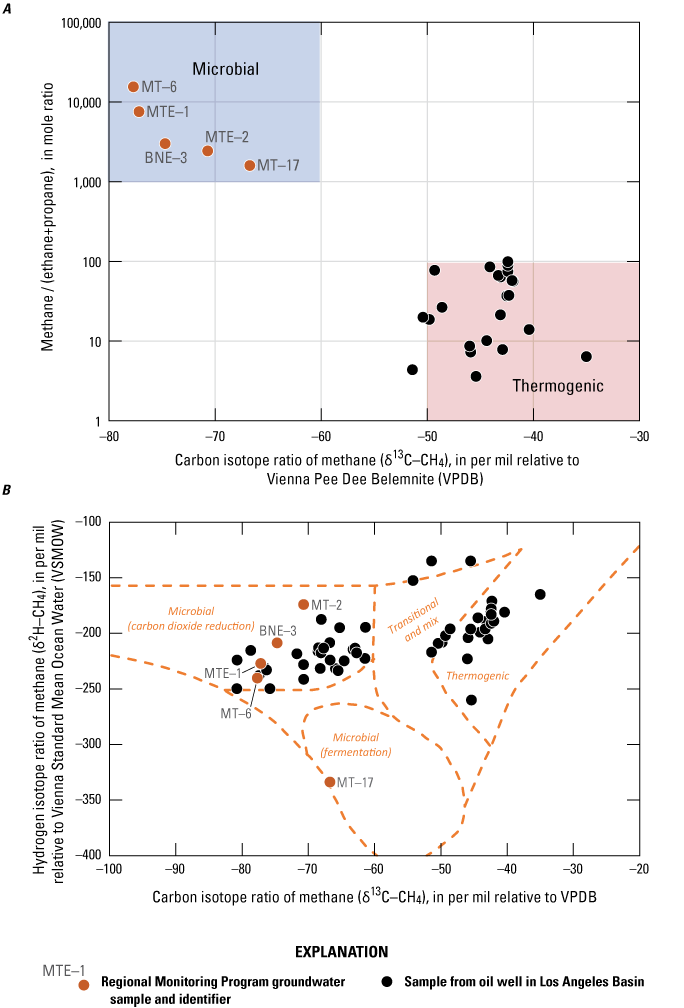
Carbon isotopic composition of methane for Regional Monitoring Program groundwater samples in the Montebello Oil Field study area, Los Angeles County, California, 2014 and 2017–18, and oil- and gas-well samples in the Los Angeles Basin (Jeffrey and others, 1991; Kulongoski and others, 2018) in relation to A, the ratio of methane to ethane plus propane; and B, the hydrogen isotopic composition of methane. Zone boundaries for microbial and thermogenic methane are from Whiticar and others (1986). Samples with methane concentrations less than 0.13 milligrams per liter are not shown because of insufficient methane in those samples to measure methane isotopes.
Methane concentrations were available from 241 historical groundwater samples (collected between 2001 and 2014) from 43 monitoring wells clustered at 10 locations (fig. 4; California State Water Resources Control Board, 2017a). All but one of those well clusters were outside the Montebello Oil Field, and none of the wells had perforation or depth information available. Methane was detected in 21 of the 43 wells. The largest methane concentration from the historical groundwater samples was 4 mg/L, collected from a well at least 0.64 km from the closest oil and gas well. The isotopic composition of methane for historical samples was not available for determining the source of methane. As discussed in the “Potential Subsurface Sources and Migration Pathways” section, thermogenic gas was detected in soils and wells in the western part of the Montebello Oil Field between the 1970s and 1990s (California Public Utilities Commission, 2001) and in oil and gas observation wells in 2018 (fig. 5; California Department of Conservation, 2020b).
Age-Dating Tracers
The age-dating tracers tritium (3H) and carbon-14 in dissolved inorganic carbon (14C-DIC) were used to determine whether groundwater samples were composed of modern-age groundwater (recharged during or after 1953), pre-modern groundwater (pre-1953), or a mixture of both. The water was classified so potential sources and pathways of VOCs could be explored: if the aquifer contained modern-age groundwater, that would indicate chemical compounds in groundwater could have originated at or near the land surface, whereas if the aquifer contained pre-modern groundwater, it would indicate that chemical compounds in groundwater originated either at the land surface before 1953 or from subsurface sources.
There were 11 samples classified as modern, 5 samples classified as pre-modern, and 5 samples that had evidence of both modern and pre-modern age water (mixed; table 3; fig. 14). Two of the samples containing only modern water (MTE-2 and BNE-2) were collected from wells that were completed with perforations that were at least 150 m bls (table 1), indicating that modern recharge can reach deep intervals in some parts of the study area within a 66-year time frame. Conversely, one of the samples containing only pre-modern water (MT-14) was collected from a relatively shallow well near the OII landfill with perforations that were less than 91 m bls (table 1). The age-tracer data from sample MT-16 are inconclusive because the sample does not contain detectable 3H (or tritiogenic helium-3 [3He]) but does contain a modern concentration of 14C-DIC (greater than 100 pmc) and manufactured VOCs (table 3; appendix 3, table 3.5). The age indicators contradict each other in that if the sample is 3H “dead,” it should be older than 66 years in age, whereas the 14C-DIC points out a modern age, which should contain 3H. This result could be explained if the water was recharged shortly before 1953. That type of water would not have detectable 3H or 14C-DIC, but 14C-DIC in recharge would still be close to 100 pmc. The contradictory result also could be caused by an analytical issue either with the 14C-DIC or 3H measurement.
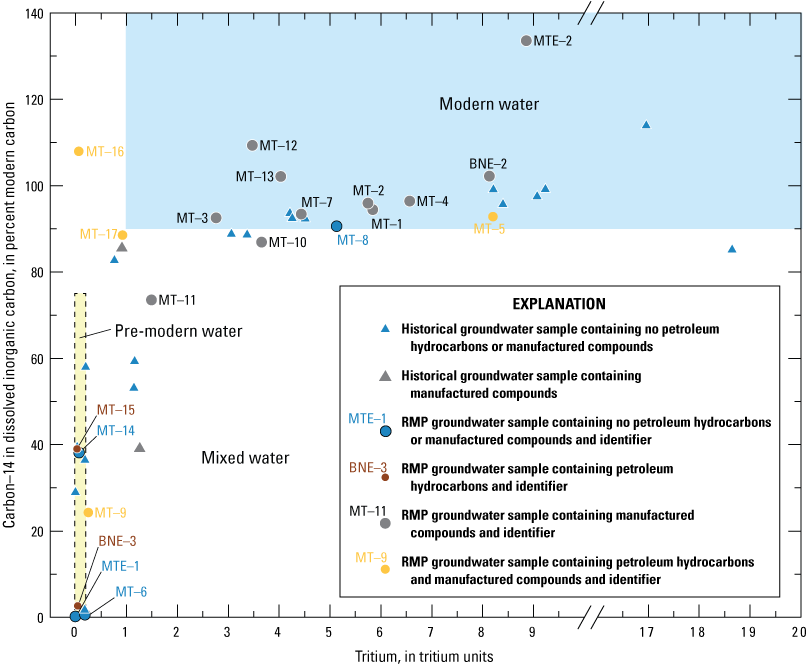
Tritium concentrations in relation to carbon-14 in dissolved inorganic carbon for Regional Monitoring Program (RMP) groundwater samples in the Montebello Oil Field study area, Los Angeles County, California, 2014 and 2017–18 and historical groundwater samples (U.S. Geological Survey, 2021).
Almost all samples (10 of 11) interpreted as containing only modern-age groundwater had detections of manufactured VOCs; petroleum hydrocarbons co-occurred in one of these samples (table 3; fig. 14). None of the five samples containing pre-modern groundwater had manufactured VOCs, but two contained petroleum hydrocarbons (table 3). All five samples with evidence of mixed-age groundwater contained manufactured VOCs, and three of those five samples contained petroleum hydrocarbons (table 3). One possible interpretation of these results is that petroleum hydrocarbons are more commonly associated with pre-modern groundwater that has not been in contact with the land surface since the 1950s, and the petroleum hydrocarbons present in samples with mixed-age groundwater are more likely to be from the pre-modern groundwater portion than from the modern portion of the sample. The four samples with co-occurring manufactured VOCs and petroleum hydrocarbons (MT-5, MT-9, MT-16, and MT-17) were collected from shallow wells (less than 70 m deep; table 1). Sample MT-16 contained many more manufactured VOCs than petroleum hydrocarbons (fig. 10), and concentrations of manufactured VOCs were larger (appendix 3, table 3.5). In the other three samples, concentrations of manufactured VOCs and petroleum hydrocarbons were generally more similar. Four samples did not have detectable petroleum hydrocarbons or manufactured VOCs: two deep samples (MTE-1 and MT-6) containing pre-modern water, a shallow sample (MT-14) with pre-modern water, and a shallow sample (MT-8) with modern water (fig. 10; table 3). The shallow sample containing only pre-modern water was collected from a well completed in confined marine sediments of the Pico Formation that are closer to the land surface than in other parts of the study area. Groundwater in those sediments may not have been flushed by modern water.
Age-dating tracer results for historical groundwater samples in the Montebello Oil Field study area indicated that modern-age groundwater has reached relatively deep intervals of at least 405 m bls at some locations and pre-modern groundwater is present at relatively shallow depths (85 m) at other locations. Tritium concentrations were available for 107 historical groundwater samples from 103 wells, including 10 of the wells sampled for this study (California State Water Resources Control Board Division of Drinking Water, 2018; Water Replenishment District of Southern California, 2019b; U.S. Geological Survey, 2021). Sample dates ranged from 1994 to 2018. Well-perforation or well-depth information was available for 102 of the 103 sampled wells. Most of the sampled wells were in the valley alluvium adjacent to the Montebello Oil Field.
There were 21 historical samples analyzed for 3H and 14C-DIC, in addition to the 21 samples collected for this RMP study area (fig. 14). Of the 42 RMP and historical samples with 3H and 14C-DIC results, 19 samples were classified as modern, 9 were pre-modern, and 14 were mixed. In general, modern groundwater was shallower than pre-modern water. Modern groundwater was collected from wells completed between 28 and 366 m (median=134 m) bls, pre-modern groundwater was from wells completed between 90 and 424 m (median=293 m) bls, and mixed groundwater was from wells completed between 53 and 424 m (median=206 m) bls. Two of the historical samples contained manufactured VOCs and were classified as mixed-age groundwater (fig. 14); petroleum hydrocarbons were not detected in these samples. There were 19 samples with no detection of either manufactured or petroleum hydrocarbon VOCs; 8 classified as modern groundwater, 4 classified as pre-modern groundwater, and 7 classified as mixed.
Inorganic Tracers
Water from hydrocarbon-bearing formations is often saline, with TDS concentrations greater than 10,000 mg/L (Richter and Kreitler, 1991; Metzger and others, 2020). The TDS concentrations in RMP samples generally increased with depth (appendix 3, table 3.7), but all except the deepest groundwater sample (BNE-3) contained freshwater (TDS less than 3,000 mg/L). The median groundwater TDS concentration was 509 mg/L. Historical groundwater data indicated freshwater at depths less than 305 m bls and a median TDS of 490 mg/L (appendix 3, table 3.7). In contrast, historical oil-field water samples collected from wells in the study area, with depths to shallowest perforation of almost 600 to more than 2,500 m bls, contained TDS concentrations ranging from about 15,000 to 30,000 mg/L (Metzger and others, 2020), with a median TDS concentration of 24,275 mg/L.
In addition to elevated TDS concentrations, water from hydrocarbon-bearing formations commonly has elevated concentrations of specific inorganic constituents, such as ammonium, barium, boron, bromide, chloride, iodide, and lithium (Angino, 1970; Compton and others, 1992; McMahon and others, 2018; Wright and others, 2019). Available historical oil-field water samples in the study area indicate that the median concentrations of these constituents were several orders of magnitude larger than RMP and historical groundwater samples (appendix 3, table 3.7). The only exception was lithium, which was not detected in three of the four oil-field water samples analyzed for lithium. However, this result is most likely related to the large LDLs for those samples. The largest concentrations of inorganic constituents in RMP and historical groundwater samples were generally identified in the deepest wells (appendix 3, table 3.7).
Chloride concentrations relative to boron, bromide, and iodide indicated that three RMP samples (MT-6, MTE-1, and BNE-3) and several historical groundwater samples (1, 2, 3, 7) have chemical characteristics that plot along an apparent mixing line between either the native groundwater and a produced water end member or between native groundwater and a seawater end member (fig. 15). The RMP samples MT-16 and MT-13 plot along an apparent mixing line between the native groundwater and imported Colorado River water for chloride relative to iodide (fig. 15A). The historical groundwater sample used to represent the native groundwater end member (U.S. Geological Survey, 1998) for the mixing line analysis was selected because it represented typical native groundwater chemistry conditions in the Central Basin. However, the large distances between decile markers indicates that the analysis is not sensitive to changes in the plotted location of the native groundwater end member (fig. 15). Samples 1, 3, and 7 (fig. 15) were collected from wells that were also sampled for the RMP (samples BNE-3, MTE-1, and MT-6, respectively), and sample 2 was collected from a well perforated from 228 to 234 m bls. The samples were collected from wells completed at depths greater than 200 m bls and perforated in marine sediments. Age-dating tracer data from the RMP samples indicate that water in those wells is pre-modern (table 3). These results indicate that some deeper wells contain naturally occurring old groundwater that has (1) interacted with marine sediments containing water with a similar composition as produced water, (2) mixed with water from subsurface hydrocarbon-bearing formations (represented by oil-field water samples), or (3) both. Previous groundwater studies in the Los Angeles Basin do not provide evidence of seawater intrusion as far inland as the Montebello Oil Field (Reichard and others, 2003; Paulinski, 2021), although paleo-seawater may be present in some marine sediments that have not been flushed with meteoric water (Piper and Garrett, 1953). Shallower samples did not have inorganic chemical characteristics associated with mixing of water from hydrocarbon-bearing or marine formations. Recycled water also composes part of the water in the study area and is a potential end member. Chloride and boron concentrations for recycled water averaged 111 mg/L and 270 µg/L (Los Angeles County Sanitation Districts, 2021), respectively, plotting in the main cluster of RMP sample results on figure 15. Therefore, recycled water is not shown as an end member on figure 15.
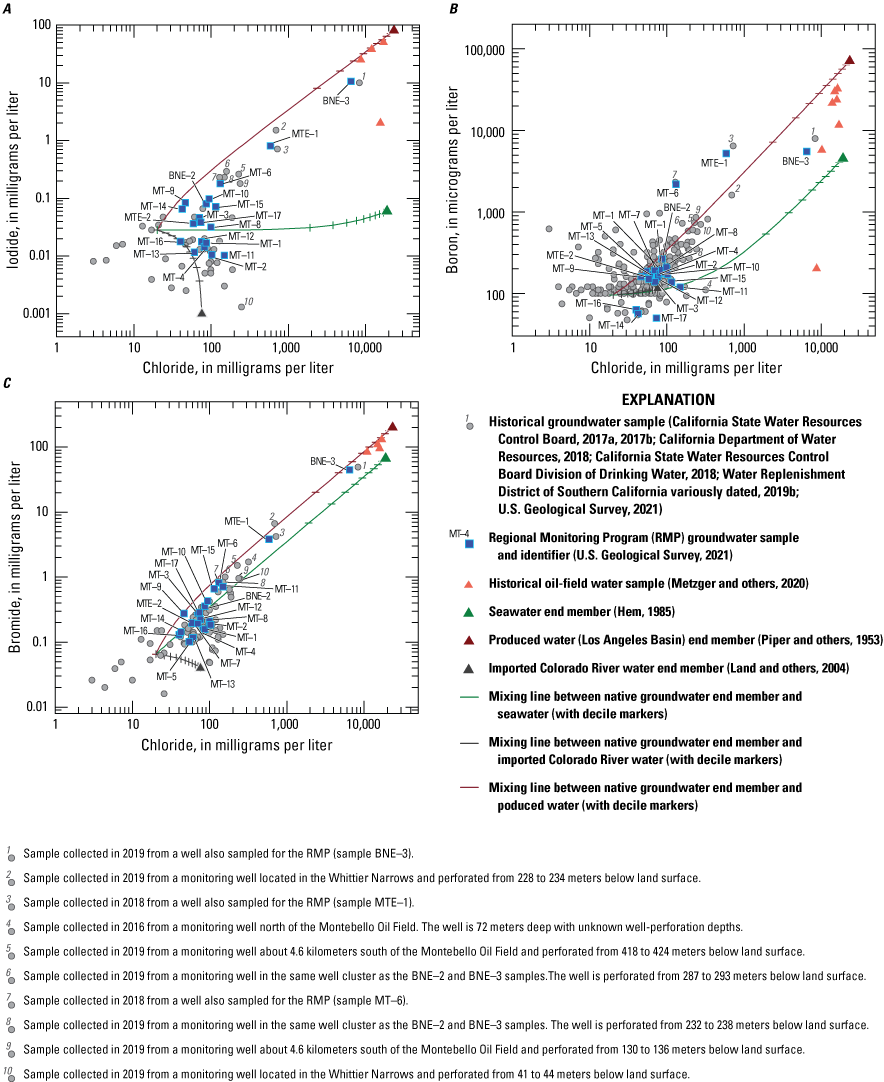
Chloride concentrations in relation to A, iodide; B, boron; and C, bromide concentrations for Regional Monitoring Program (RMP) groundwater samples in the Montebello Oil Field study area, Los Angeles County, California, 2014 and 2017–18, historical groundwater samples, and historical oil-field water samples. Locations of labeled historical groundwater samples that were not collected from wells sampled for the RMP are shown on figure 10.
The relative proportions of major inorganic constituents as charge equivalents can be useful for identifying groundwater origins and interactions (Appelo and Postma, 1999). A Piper diagram was used to show the relative contribution of major cations and anions, on a charge-equivalent basis, to the total ionic content of the water (Piper, 1944). Historical oil-field water chemistry samples in the study area generally clustered and had the highest relative proportions of sodium and chloride (fig. 16) compared to groundwater samples. Most groundwater samples collected for the RMP study are geochemically similar to most of the historical groundwater samples. Exceptions to this pattern are samples from the three deepest wells (MT-6, MTE-1, and BNE-3) and a shallow monitoring well perforated from 64 to 67 m bls (MT-9; table 1). Sample BNE-3 was most geochemically similar to the oil-field water samples and seawater and also was the deepest sample collected as part of the RMP (perforated from 405 to 424 m bls). Concentrations of TDS and many inorganic constituents in sample BNE-3 were greater than most other groundwater samples but less than in oil-field water (fig. 15; Metzger and others, 2020; U.S. Geological Survey, 2021). Samples MT-6, MT-9, and MTE-1 have higher proportions of sodium and bicarbonate and smaller proportions of calcium, magnesium, chloride, and sulfate than most groundwater samples (fig. 16). Their composition is consistent with cation exchange of calcium and magnesium for sodium, calcite precipitation, or sulfate reduction (Appelo and Postma, 1999). This pattern is similar to groundwater that has moved deeper into the flow system in the coastal California aquifers (Izbicki and others, 2003). Samples MT-6 and MTE-1 contain pre-modern water and elevated concentrations of TDS, ammonium, and many other inorganic constituents. Sample MT-9 was collected from a shallow well containing a mixture of modern and pre-modern groundwater (table 3) as well as relatively high concentrations of several trace elements (aluminum, arsenic, fluoride, lithium, and molybdenum), high pH, and relatively low concentrations of TDS. This sample is from a monitoring well in the San Gabriel Valley Superfund area (fig. 6) and its unique chemical composition could be affected by upgradient contamination and remediation activities. The RMP samples composed of modern water have a mixed cation and anion signal that is consistent with younger groundwater that has not extensively interacted with aquifer sediments (fig. 16). Those samples were similar in composition to most of the historical groundwater samples.
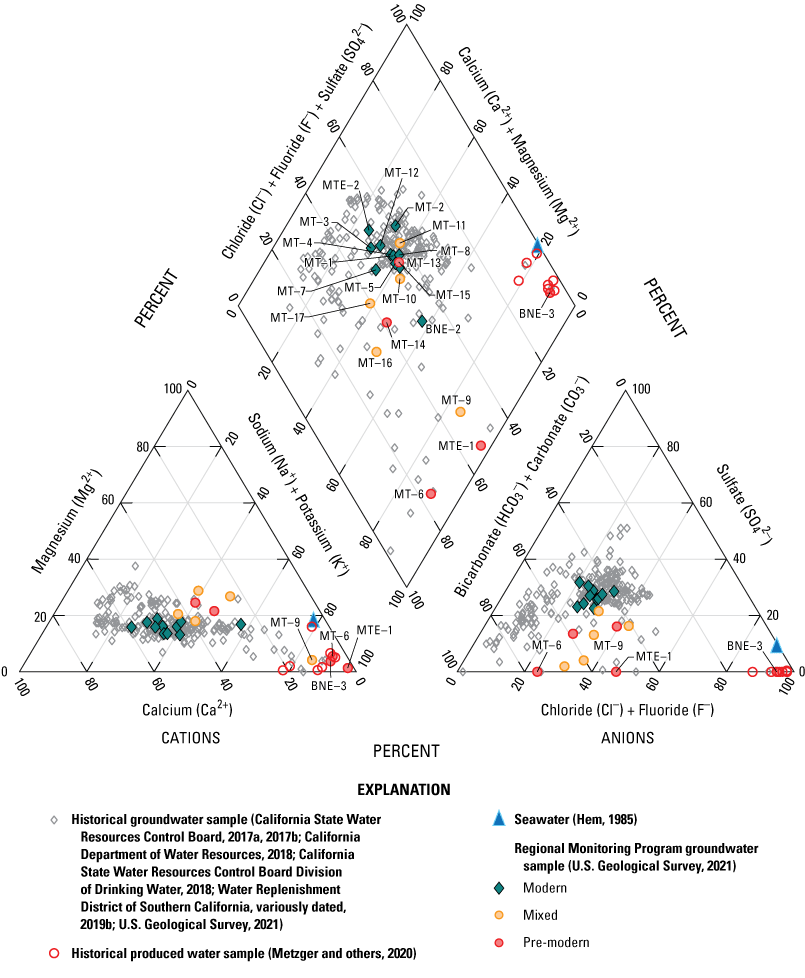
Relative proportions, in percentage equivalents, of major ions by age category for Regional Monitoring Program groundwater samples in the Montebello Oil Field study area, Los Angeles County, California, 2014 and 2017–18, historical groundwater samples, and historical oil-field water samples.
Geochemical Results in Relation to Water-Quality Benchmarks
The groundwater samples discussed in this report were compared to water-quality benchmarks to provide a frame of reference for the measured concentrations. Note that some groundwater samples were collected from monitoring or irrigation wells not used as a source of drinking water, and raw water collected from groundwater pumped from wells used for drinking-water supply undergoes treatment and blending before being delivered to consumers. Consequently, none of the samples discussed directly characterize drinking-water quality. These results cannot be firmly linked to hydrocarbon-bearing formations but provide a basis for understanding the relative magnitude of chemical constituents in RMP samples.
Chemical results for seven of the RMP samples indicated that at least one constituent did not meet enforceable U.S. Environmental Protection Agency (2018) and California State Water Resources Control Board (2019c) standards and health advisories (appendix 3, table 3.8). Turbidity was the only constituent that did not meet its standard in four of those seven samples. Of the samples, 67 percent (14 of 21) contained at least one chemical constituent at a concentration greater than nonenforceable drinking-water benchmarks (U.S. Environmental Protection Agency, 2018; California State Water Resources Control Board, 2019c) or USGS health-based screening levels (U.S. Geological Survey, 2018), which were created to ensure that drinking water does not have undesirable cosmetic or aesthetic effects (appendix 3, table 3.8). Most of the concentrations that were greater than benchmarks were for inorganic constituents. The only organic constituents present at concentrations greater than benchmark standards were the solvents tetrachloroethene (PCE; in sample MT-16), trichloroethene (TCE; in samples MT-12 and MT-16), and cis-1,2-dichloroethene (in sample MT-16). Sample MT-16 was collected from a monitoring well near the OII landfill (fig. 6), and sample MT-12 was untreated water collected from a public-supply well in the Bandini Oil Field (fig. 1). Large concentrations of PCE and TCE are consistent with previously published results for the Coastal Groundwater Basin (Shelton and others, 2001; Fram and Belitz, 2012) and with available historical data in the study area (California State Water Resources Control Board, 2017a, 2017b; California State Water Resources Control Board Division of Drinking Water, 2018; Water Replenishment District of Southern California, variously dated, 2019b; U.S. Geological Survey, 2021).
Potential Sources and Migration Pathways of Chemical Constituents
Important potential sources and transport pathways associated with oil and gas development that could affect groundwater quality in the Montebello Oil Field include large volumes of recycled produced water that have been reinjected since the 1960s to enhance oil production (California Department of Conservation, variously dated; California Department of Conservation, 1992), old oil and gas wells that may be more likely to develop well-integrity issues than newer wells, and oil and gas wells with an uncemented annulus interval that intersects groundwater zones (California Department of Conservation, 2020c). The intersection of areas with high net injection (shown as a positive change in fluid height on fig. 8) and reservoir pressure in the eastern and southern part of the oil field with the presence of wells that have mechanical integrity issues (appendix 3, table 3.1) or uncemented annuli that intersect groundwater zones (fig. 9) increases risk for migration of water from oil-bearing formations. Oil and gas development also can affect groundwater from surficial sources, such as disposal ponds, well sumps, and other infrastructure at the land surface (fig. 6; Entrix, Inc., 2009).
Although potential sources and pathways exist, the direction and magnitude of hydraulic gradients to drive fluid movement vertically are generally unknown. Consequently, the occurrence, duration, and magnitude of upward water or gas fluxes through leaky oil and gas wells to groundwater are unknown. In addition, water flooding in the Montebello Oil Field involves recycled produced water, and the chemical composition of injection water is similar to formation water. Therefore, distinguishing among leaks from injection water, mixtures of injection water with oil- and gas-producing zone fluids, migrated oil- and gas-producing zone fluids, and natural geologic processes before oil development is difficult. The losses of fluids from target zones during the installation and development of injection and production wells have been noted in other cases outside of those reported but have not been systematically documented across the field; the magnitude of these subsurface releases is unknown. A connection between water quality in groundwater wells and nearby oil and gas wells with possible integrity issues cannot be ruled out or conclusively established; additional details are discussed on a well-by-well basis in this section.
The RMP sample results and available historical data (California State Water Resources Control Board, 2017a, 2017b; California Department of Water Resources, 2018; California State Water Resources Control Board Division of Drinking Water, 2018; Water Replenishment District of Southern California, variously dated, 2019b; U.S. Geological Survey, 2021) in the Montebello Oil Field study area provide no conclusive evidence that oil and gas development or natural flow from hydrocarbon-bearing formations have affected groundwater quality, beyond those local cases noted historically (Entrix, Inc., 2009; California Public Utilities Commission, 2001). Numerous surficial sources of hydrocarbons have been documented near and in the Montebello Oil Field, and all RMP samples with detectable petroleum hydrocarbons, thermogenic gas, or both, were collected from sites within 500 m of anthropogenic surficial sources not associated with oil and gas development. In addition, natural sources of shallow hydrocarbons could affect groundwater quality.
Dissolved petroleum hydrocarbons or thermogenic gas were detected at low concentrations in 7 of the 21 RMP groundwater samples (table 4). Five of those samples (MT-5, MT-9, MT-10, MT-16, and MT-17) also contained manufactured VOCs and at least some modern-age groundwater, indicating that the hydrocarbons could have originated from surficial or shallow sources unrelated to oil and gas development. Two samples (MT-15 and BNE-3) contained low concentrations of petroleum hydrocarbons (0.015 and 0.0186 µg/L, respectively) and did not contain detectable concentrations of manufactured VOCs in pre-modern groundwater (appendix 3, table 3.5). These samples were collected from intermediate and deep wells, respectively, with perforations completed in marine sediments (table 1) that may contain water with similar compositions to oil-field water (fig. 16). Thermogenic gases in the propane through pentane range were detected in four samples (MT-10, MT-15, MT-16, and MT-17; table 4; appendix 3, table 3.6), indicating at least a small fraction of the gases at these sites are derived from thermogenic sources. Potential sources and pathways of chemical constituents for each of the seven samples containing petroleum hydrocarbons or thermogenic gas are discussed in this section.
Table 4.
Conceptual summary of detections of different classes of compounds, groundwater age, and inferred observations on sources of constituents for Regional Monitoring Program groundwater samples in the Montebello Oil Field study area, Los Angeles County, California, 2014 and 2017–18 (U.S. Geological Survey, 2021).Sample identifier (fig. 1) |
Dissolved petroleum hydrocarbons detected |
Thermogenic hydrocarbon gas detected |
Groundwater- age classification |
Manufactured volatile organic compounds detected |
Inorganic chemistry indicates mixing with water from oil-bearing or marine formations |
Possible hydrocarbon source(s) |
Location |
---|---|---|---|---|---|---|---|
MTE-1 | No | No | Pre-modern | No | Yes | None. Possible water from oil-bearing or marine formations. | Southwest of Montebello Oil Field |
BNE-3 | Yes | No | Pre-modern | No | Yes | Deep source likely. | Bandini Oil Field |
MT-5 | Yes | No | Modern | Yes | No | Surface source likely. | San Gabriel Valley Superfund area |
MT-6 | No | No | Pre-modern | No | Yes | None. Possible water from oil-bearing or marine formations. | Southwest of Montebello Oil Field |
MT-9 | Yes | No | Mixed | Yes | No | Surface source likely. | San Gabriel Valley Superfund area |
MT-10 | No | Yes | Mixed | Yes | No | Surface source likely, deep source possible. | Bandini Oil Field |
MT-15 | Yes | Yes | Pre-modern | No | No | Surface source possible, deep source likely. | Montebello Oil Field Gas Storage area, Operating Industries Inc. (OII) Superfund area |
MT-16 | Yes | Yes | Mixed | Yes | No | Surface source likely. | Operating Industries Inc. (OII) Superfund area |
MT-17 | Yes | Yes | Mixed | Yes | No | Surface source likely, deep source possible. | North of Montebello Oil Field |
Sample MT-10 contained eight manufactured VOCs and did not contain petroleum hydrocarbons but did have a small concentration of the thermogenic gas propane (0.0001 mg/L) (table 4; appendix 3, tables 3.5, 3.6). The sample was collected from a well with perforations that span shallow and intermediate depths and was a mixture of modern and pre-modern groundwaters (tables 1, 4); therefore, the thermogenic gas could be from water entering the well at deeper intervals, and the manufactured VOCs could be from water entering at shallow intervals. Sample MT-10 is in the Bandini Oil Field within 500 m of four oil and gas wells (fig. 1) all drilled and abandoned before 1976, including one well with a noted integrity issue (table 2; appendix 3, table 3.1). Sample MT-10 also is within 500 m of two State Water Board Cleanup Sites that were established to remediate petroleum hydrocarbons in soil (fig. 6; California State Water Resources Control Board, 2019b). The inorganic chemical composition of MT-10 is closer in composition to most of the historical groundwater samples than to oil-field water samples (figs. 15, 16) and is not consistent with the presence of water from hydrocarbon-bearing formations; however, small amounts of thermogenic gas migration from hydrocarbon-bearing formations independent of water movement cannot be ruled out.
Samples MT-5, MT-9, MT-15, and MT-16 were collected from locations in EPA Superfund areas with known hydrocarbon contaminants (fig. 6; U.S. Environmental Protection Agency, 2011, 2015). Samples MT-5 and MT-9 were collected from two shallow monitoring wells (table 1) located next to each other on the northeast side of the Montebello Oil Field near the edge of the San Gabriel Valley Superfund area (established to remediate VOCs originating from numerous upgradient sources). Samples MT-5 and MT-9 had modern- and mixed-age water, respectively (table 3). Sample MT-5 contained benzene, PCE, and trichloromethane at concentrations less than 0.04 µg/L, and sample MT-9 contained benzene and PCE at concentrations less than 0.03 µg/L (appendix 3, table 3.5). Historical groundwater samples collected between 2001 and 2003 from the MT-5 well (as part of the superfund monitoring activities) had large concentrations of PCE (greater than 100 µg/L; U.S. Environmental Protection Agency, 2006), indicating that the well had been affected by industrial sources. These results, along with information about regional groundwater-flow directions (fig. 1), indicate that VOCs detected in MT-5, including petroleum hydrocarbons, are likely from sources upgradient from the Montebello Oil Field. Also, PCE was not detected in historical groundwater samples collected from well MT-9 during the same 2001–03 period. However, the PCE LDL used for the superfund area analyses was about an order of magnitude higher than was used for the RMP analysis, and PCE could have been present in historical samples if a smaller LDL had been used. Nevertheless, the MT-5 and MT-9 wells are upgradient from the Montebello Oil Field (fig. 1).
Samples MT-15 and MT-16 were collected downgradient from the OII landfill Superfund area (U.S. Environmental Protection Agency, 2020b; fig. 6). Sample MT-15 was from an intermediate depth well (table 1) within 500 m of 95 oil and gas wells and the Montebello gas storage field project area (fig. 5). Two petroleum hydrocarbons (1,2,4-trimethylbenzene and 4-isopropyl-toluene) and one thermogenic gas (isopentane) were detected at small concentrations (appendix 3, tables 3.5, 3.6); the petroleum hydrocarbon concentrations were smaller than laboratory detection levels (appendix 3, tables 3.4, 3.5). Inorganic tracers in this sample are closer in composition to most of the historical groundwater samples than to oil-field water samples (figs. 15, 16) and are not consistent with the presence of water from hydrocarbon-bearing formations. Most fluid injection activities are more than 1 km to the east, although one injection well (American Petroleum Institute [API] well identifier 0403721483, California Department of Conservation, 2019b) used for water flooding is within about 140 m. That well injected about 0.2 million m3 between 1977 and 2018, at depths greater than 1,900 m (California Department of Conservation, 2020c). Based on available records, nine of the nearby oil and gas wells had reported well-integrity issues at depths potentially intersecting groundwater, two wells had surface expressions of oil, water, or gas leaks with unknown intersection with groundwater, and nine wells had integrity issues within deeper oil- and gas-producing zones unlikely to affect groundwater (table 2; appendix 3, table 3.1). Thermogenic gas has been previously detected in soils, abandoned wells, and monitoring wells in the Montebello gas storage field project area (fig. 5), which includes MT-15 (see the “Potential Subsurface Sources and Migration Pathways” section), but the small concentration of isopentane in this sample indicates that thermogenic gas is insubstantial at this site. The intermediate depth well perforations and absence of evidence of modern-age water (from age-dating tracers or the presence of manufactured VOCs) indicates the trace detections of petroleum hydrocarbons and thermogenic gas in this sample did not originate from a source near land surface and could be naturally occurring, affected by subsurface oil and gas development, or both. Sample MT-16 was collected from a shallow monitoring well and contained 12 manufactured VOCs, 5 petroleum hydrocarbons, and 4 thermogenic gases (propane through n-pentane; appendix 3, tables 3.5, 3.6). Concentrations of the manufactured VOCs PCE, TCE, and cis-1,2-dichloroethene were greater than their respective drinking-water benchmarks (U.S. Environmental Protection Agency, 2018; California State Water Resources Control Board, 2019c). The presence of many manufactured VOCs of multiple types indicates that landfill activities are a likely source of petroleum hydrocarbons in this sample. This sample contained water with mixed groundwater ages; therefore, a modern fraction of groundwater near surface contaminants could potentially influence this sample.
Sample MT-17 was collected from a shallow, inactive irrigation well about 1-km north of the Montebello Oil Field administrative boundary near several possible sources of contaminants (fig. 6). The sample contained two manufactured VOCs, four petroleum hydrocarbons at concentrations less than 0.05 µg/L, and three thermogenic gases (propane through n-pentane; appendix 3, tables 3.5, 3.6). Inorganic tracers in this sample are not consistent with the presence of water from hydrocarbon-bearing formations (figs. 15, 16). The well is about 200 m south of a former underground storage tank that had leaked diesel fuel and within 750 m of two additional State Water Board Cleanup Sites established to remediate petroleum hydrocarbons (California State Water Resources Control Board, 2019b). The well also is about 180 m northwest of two oil and gas wells, with reported well-integrity issues intersecting the groundwater resource zone (appendix 3, table 3.1; California Department of Conservation, 2020c). One of those wells (API well identifier 0403705134, California Department of Conservation, 2019b) was a dry hole abandoned in 1921 without a cement plug (appendix 3, table 3.1). The other well (API well identifier 0403705135, California Department of Conservation, 2019b) has been unused since 1924. The casing of well 0403705135 had collapsed at 221 m bls and filled with water to within 12 m bls. An abandonment proposal was filed for this oil well in 1924, but abandonment activities have not been documented in online records. The sampled irrigation well and the two abandoned oil and gas wells are situated near a fault line (fig. 5), which has unknown potential to be a natural pathway for migration of fluids from hydrocarbon-bearing formations. The MT-17 sample also is near the western edge of the San Gabriel Valley Superfund area (fig. 6). The 3H and 14C-DIC age-dating tracers indicate the MT-17 sample contains a mixture of modern and pre-modern groundwater (fig. 14). Data were not available to determine the direction of groundwater flow near this well. Based on these data, the source(s) that may have contributed petroleum hydrocarbons to this sample are unknown.
Sample BNE-3 was collected from the deepest well used for the RMP in the study area (table 1). The well is downgradient from the Montebello Oil Field, in the Bandini Oil Field (fig. 5). This sample had inorganic chemical characteristics most like historical oil-field water samples (figs. 15, 16). Sample BNE-3 contained one petroleum hydrocarbon (benzene concentration of 0.015 µg/L; appendix 3, table 3.5) and elevated concentrations of dissolved solids, chloride, ammonium, barium, lithium, boron, alkalinity, iron, iodide, bromide, and dissolved organic carbon relative to other RMP samples and historical groundwater data. However, this sample did not have any heavier gases or thermogenic methane (appendix 3, table 3.6). Historical records for the 19 oil and gas wells within 500 m of this sample indicate that petroleum hydrocarbons were observed within about 90 vertical m below the BNE-3 sample interval (table 1; appendix 3, table 3.3), and one well (API well identifier 0403700819, California Department of Conservation, 2019b) had known well-casing damage at depths of 977, 1,164–1,166, and below 1,252 m bls (appendix 3, table 3.1). Conditions at some oil and gas wells were favorable for upward movement of water, based on idle well fluid-level elevations (appendix 3, table 3.3) close to or higher than the groundwater level measured in the RMP sampled well (table 1). Although the idle well fluid-level data have substantial uncertainties, these results indicate that head gradients could be upward at this location. However, contemporaneously measured groundwater levels and idle well fluid levels would be needed to assess vertical gradients between oil- and gas-producing zones and overlying groundwater, and the patterns observed herein are preliminary assessments. Several faults transect the Bandini Oil Field (fig. 5) and have unknown potential to be a natural pathway for migration of fluids from hydrocarbon-bearing formations (California Department of Conservation, 1992). Overall, the chemical composition of the BNE-3 sample could be a result of naturally occurring processes, migration of water from hydrocarbon-bearing formations through human-caused pathways, or both.
Study Limitations and Additional Analysis
The initial phase of the RMP uses existing wells to determine groundwater conditions across many oil field areas in California. As a result, data used in this study to determine potential sources and migration pathways of chemical constituents that could affect local groundwater quality were non-ideal and limited by the small number of wells available in the Montebello Oil Field study area. Due to the low density of RMP and historical data (California State Water Resources Control Board, 2017a, 2017b; California Department of Water Resources, 2018; California State Water Resources Control Board Division of Drinking Water, 2018; Water Replenishment District of Southern California, variously dated, 2019b; U.S. Geological Survey, 2021), particularly in parts of the study area with the largest potential risks to groundwater, a definitive determination of the effects of nearby anthropogenic activities and natural factors on groundwater quality was not possible. Thus, the conclusion that oil and gas development has not negatively affected groundwater resources in this study area was influenced by the limited information available.
Areas to consider for more detailed monitoring and analysis in the future that may present the largest relative potential risks to groundwater quality include (1) areas downgradient from historical surface ponds and sumps and (2) areas with co-located high net injection (oil reservoir injection exceeds production), old oil and gas wells that may be more likely to develop well-integrity issues than newer wells, and oil and gas wells with uncemented boreholes intersecting groundwater zones.
Completion of several activities could help evaluate the potential effects of oil and gas development and other industrial processes in the area on local groundwater resources, particularly in areas of relatively greater risk mentioned earlier:
-
additional groundwater sampling at various vertical and horizontal distances from oil and gas production;
-
data collection regarding compositions of potential geochemical end members, including oil- and gas-producing zones and samples from discrete depths where oil and gas shows in aquifer sediments overlying the zones of oil and gas production occur;
-
data collection and analysis to determine pressure gradients between oil production and groundwater zones and their relations to groundwater withdrawals and oil and gas production and injection;
-
expanded analysis of oil and gas well cement seals across the oil field, including the vertical distribution of cement in the annular space around the well casing, historical records of recorded well-integrity barrier failures (a breach in a protective system), and well-integrity failures (a breach in a protective system resulting in a documented leak) to document the incidence of potential pathways; and
-
analysis of available temperature and borehole resistivity data, which are available in some areas of oil and gas development where groundwater wells are absent and can be used to identify anomalous observations that could be consistent with movement of heated or saline water from hydrocarbon-bearing formations into groundwater.
Summary
Groundwater quality and potential sources and migration pathways of chemical constituents associated with hydrocarbon-bearing formations were assessed by the U.S. Geological Survey (USGS) for the California State Water Resources Control Board Oil and Gas Regional Monitoring Program (RMP). The Montebello Oil Field was selected by the State Water Board, in consultation with the Los Angeles Regional Water Quality Control Board, as an assessment priority. This selection was based on historical oil development and gas storage activities in the field and proximity of the field to significant natural and enhanced regional groundwater recharge areas for the Central Basin, where groundwater wells supply water to meet the needs of millions of people. Relative to the approximately 500 onshore oil fields in California, the Montebello Oil Field has a high volume of water injected into the field, a high density of oil and gas wells, and a moderate vertical proximity of petroleum development and groundwater resources.
Groundwater samples were collected from 21 preexisting wells by the RMP between 2014 and 2018. These wells were thought to be potentially affected by oil and gas development and supplemented with historical groundwater chemistry data from other USGS and State sources. Samples were analyzed for a wide range of chemical constituents to identify potential sources of petroleum hydrocarbons, gases, and inorganic constituents associated with hydrocarbon-bearing formations.
Important potential sources and migration pathways that could affect groundwater quality in the study area include (1) large volumes of recycled produced water that have been reinjected since the 1960s to enhance oil production (which could increase reservoir pressures and drive fluids out of oil- and gas-producing zones) and (2) oil and gas wells that intersect groundwater zones and have well-integrity issues, an uncemented annulus interval, or both, that create pathways for fluid migration from deeper intervals into shallower aquifers. The risk of changes to groundwater quality from petroleum hydrocarbons and other chemical constituents increases where high net injection and reservoir pressures are co-located with the presence of wells that intersect groundwater zones and have mechanical integrity issues, uncemented annuli, or both. Oil and gas development, such as disposal ponds, well sumps, and other infrastructure at the land surface, also can affect groundwater quality via infiltration of chemical constituents to unconfined aquifers.
The Montebello Oil Field is in an urban setting dominated by commercial, industrial, and high-density residential land use that has potential to affect groundwater quality. Historical records note that petroleum hydrocarbons and thermogenic gas related to oil and gas development were found in soils and groundwater in the Montebello Oil Field. Previous studies also have documented soil and groundwater contamination associated with activities unrelated to oil and gas development in areas near the Montebello Oil Field. These activities are primarily from nearby U.S. Environmental Protection Agency Superfund areas that have affected groundwater quality throughout large areas and, in smaller areas, leaking underground storage tanks and other State Water Board Cleanup Sites established to remediate petroleum hydrocarbons and other chemical constituents.
Petroleum hydrocarbons were detected in six (29 percent) RMP groundwater samples at concentrations more than 10 times smaller than drinking-water benchmarks. None of the identified petroleum hydrocarbons exceeded 0.1 micrograms per liter (µg/L), and only 5 of the 14 compounds detected were equal to or larger than their respective laboratory detection levels (LDLs). Manufactured volatile organic compounds (VOCs) were detected in RMP samples more often (71 percent of RMP samples) and at larger concentrations (0.013–19.6 µg/L) than petroleum hydrocarbons. However, the concentrations of most manufactured VOCs were well below drinking-water benchmarks and many were smaller than LDLs. Most of the identified, manufactured VOCs were solvents. The frequent detection of manufactured VOCs is likely related to the long-term urban development of the area and indicates that groundwater quality has been affected to a greater degree by anthropogenic activities unrelated to oil and gas development.
Dissolved methane was detected in 18 RMP samples, at concentrations ranging from 0.0009 to 110 milligrams per liter (mg/L), with a median of 0.0085 mg/L. Results from the five samples with a methane concentration large enough for isotopic analysis of hydrogen and carbon indicated methane originated from microbial sources and not from deep, oil- and gas-producing zones of the Montebello Oil Field. However, heavier gases in the propane through pentane (C3–C5) range also were detected in four of the samples that contained methane, indicating at least a small fraction of the gases at these sites are derived from thermogenic sources. Three of these samples also contained petroleum hydrocarbons. Concentrations of these gases were small compared to the total volume of dissolved gas (0.0034 mole percent or less).
All except the deepest groundwater sample contained freshwater (total dissolved solids [TDS] less than 3,000 mg/L), and TDS concentrations in RMP samples generally increased with depth. Chloride concentrations relative to boron, bromide, and iodide concentrations indicated that the three deepest samples have chemical characteristics, indicating that deeper intervals either contain naturally occurring old groundwater that has interacted with marine sediments or contain old water that has mixed with water from hydrocarbon-bearing formations. Shallower samples did not have inorganic chemical characteristics associated with water from hydrocarbon-bearing formations.
Dissolved petroleum hydrocarbons or thermogenic gas were detected at low concentrations in 7 of the 21 RMP groundwater samples. Five of those samples also contained manufactured VOCs and at least some modern-age groundwater, indicating that the hydrocarbons could have originated from surficial or shallow sources unrelated to oil and gas development. Two samples contained low concentrations of petroleum hydrocarbons (0.015–0.0186 µg/L) and did not contain manufactured VOCs in pre-modern groundwater. These samples were collected from relatively deep wells with perforations completed in marine sediments that may contain water with chemically similar compositions to produced water.
Sample BNE-3 was the deepest sample collected for the RMP in the study area and was collected from a groundwater monitoring well in the nearby Bandini Oil Field. The sample had chemical characteristics most similar to historical produced water samples. Sample BNE-3 contained one petroleum hydrocarbon (benzene concentration of 0.015 µg/L) and elevated concentrations of TDS and other inorganic constituents relative to other RMP samples and historical groundwater data. Historical records for the 19 oil and gas wells within 500 m of well BNE-3 indicated that petroleum hydrocarbons were observed about 90 vertical m below the well interval. Of the 19 oil and gas wells, 1 well had known well-casing construction integrity problems. Additionally, fluid levels in a minority of idle oil and gas wells near this sample were close to or higher than the groundwater level measured in the RMP sampled well, indicating upward head gradients between oil reservoirs and groundwater may happen at some locations and times. However, these results are based on limited data; more comprehensive data on vertical head differences would help confirm this hypothesis. Overall, the chemical composition of this sample could be a result of naturally occurring processes, migration of water from hydrocarbon-bearing formations through human-caused pathways, or both.
The RMP groundwater sample results and available historical data in the Montebello Oil Field study area did not provide conclusive evidence that anthropogenic oil field activities or natural flow from oil- and gas-producing zones have affected groundwater quality, beyond those local cases noted historically. Numerous surficial sources of hydrocarbons have been documented in the Montebello Oil Field study area; however, all RMP samples with detectable petroleum hydrocarbons or thermogenic gas were collected from sites within 500 meters (m) of anthropogenic surficial sources (not associated with oil and gas development). In addition, natural sources of hydrocarbons that are present in formations outside of oil- and gas-producing zones could affect groundwater quality.
The low density of RMP and historical data, particularly in parts of the study area with the largest potential risks to groundwater, prevented a definitive analysis of relations of groundwater quality to potential anthropogenic and natural explanatory factors. Thus, the conclusion that oil and gas development has not negatively affected groundwater resources in this study area was influenced by the limited information available. Conclusions could be strengthened or refuted if more detailed monitoring and analyses are completed in areas that may present the largest relative potential risks to groundwater quality, including (1) areas downgradient from historical surface ponds and sumps and (2) areas with co-located high net injection (oil reservoir injection exceeds production), old oil and gas wells that may be more likely to develop well-integrity issues than newer wells, and oil and gas wells with uncemented boreholes intersecting groundwater zones.
References Cited
Agency for Toxic Substances and Disease Registry, 1996, Carbon disulfide CAS# 75-15-0: Agency for Toxic Substances and Disease Registry, Public Health Statement, 4 p., accessed July 31, 2020, at https://www.atsdr.cdc.gov/ToxProfiles/tp82-c1-b.pdf.
Anders, R., Landon, M.K., McMahon, P.B., Kulongoski, J.T., and Hunt, A.G., 2022, Occurrence of water and thermogenic gas from oil-bearing formations in groundwater near the Orcutt Oil Field, California, USA: Journal of Hydrology-Regional Studies, v. 41, 20 p. [Available at https://doi.org/10.1016/j.ejrh.2022.101065.]
Angino, E.E., 1970, Selective element recovery from oil field brines: Water Resources Research, v. 6, no. 5, 4 p. [Available at https://doi.org/10.1029/WR006i005p01501.]
Blondes, M.S., Gans, K.D., Engle, M.A., Kharaka, Y.F., Reidy, M.E., Saraswathula, V., Thordsen, J.J., Rowan, E.L., and Morrissey, E.A., 2017, U.S. Geological Survey National Produced Waters Geochemical Database v2.3 (PROVISIONAL): U.S. Geological Survey, accessed February 16, 2018, at https://energy.usgs.gov/EnvironmentalAspects/EnvironmentalAspectsofEnergyProductionandUse/ProducedWaters.aspx#3822349-data.
Brantley, S.L., Yoxtheimer, D., Arjmand, S., Grieve, P., Vidic, R., Pollak, J., Llewellyn, G.T., Abad, J., and Simon, C., 2014, Water resource impacts during unconventional shale gas development—The Pennsylvania experience: International Journal of Coal Geology, v. 126, p. 140–156. [Available at https://doi.org/10.1016/j.coal.2013.12.017.]
California Council on Science and Technology, 2015, Well stimulation in California (SB4): California Council on Science and Technology web page, accessed June 12, 2017, at https://ccst.us/projects/hydraulic_fracturing_public/SB4.php.
California Department of Conservation, 1992, California oil and gas fields volume II—Southern, central coastal, and offshore California oil and gas fields: California Department of Conservation Oil and Gas Technical Report, 645 p., accessed March 29, 2022, at https://www.conservation.ca.gov/calgem/pubs_stats/Pages/technical_reports.aspx.
California Department of Conservation, 2007, Montebello Field rules: California Department of Conservation web page, Geologic Energy Management Division Field Rules web page, accessed May 24, 2021, at https://www.conservation.ca.gov/calgem/field_rules.
California Department of Conservation, 2018, All wells spatial data for oil and gas well locations and associated records: California Department of Conservation web page, accessed April 2018 at https://www.conservation.ca.gov/calgem/maps/Pages/GISMapping2.aspx.
California Department of Conservation, 2019a, California statutes and regulations for the Division of Oil, Gas, and Geothermal Resources—April 2019: California Department of Conservation web page, accessed January 2020 at https://www.conservation.ca.gov/calgem/Pages/Oil,-Gas,-and-Geothermal-Rulemaking-and-Laws.aspx.
California Department of Conservation, 2019b, Well search: California Department of Conservation web page, accessed December 2019 at https://secure.conservation.ca.gov/WellSearch.
California Department of Conservation, 2020a, Well finder: California Department of Conservation web page, accessed December 21, 2020, at https://maps.conservation.ca.gov/doggr/wellfinder/#/-117.97631/33.98750/11.
California Department of Conservation, 2020b, Data for well 0403721914: California Department of Conservation, Geologic Energy Management Division web page, accessed March 29, 2022, at https://wellstar-public.conservation.ca.gov/Well/Well/Index.
California Department of Conservation, 2020c, Oil and gas online data: California Department of Conservation, Geologic Energy Management Division web page, accessed January 8, 2020, at https://www.conservation.ca.gov/calgem/Online_Data/Pages/Index.aspx.
California Department of Conservation, 2021, Well stimulation disclosure search: California Department of Conservation, Geologic Energy Management Division web page, accessed September 14, 2021, at https://wellstar-public.conservation.ca.gov/WellStimulation/WellStimulation/Index.
California Department of Conservation, variously dated, Summary of operations annual reports: California Department of Conservation web pages, accessed April 20, 2020, at ftp://ftp.consrv.ca.gov/pub/oil/Summary_of_Operations/ and ftp://ftp.consrv.ca.gov/pub/oil/annual_reports/.
California Department of Water Resources, 1961a, Effects of waste water disposal, Fruitvale Oil Field, Kern County: California Department of Water Resources and U.S. Geological Survey, 150 p., accessed December 15, 2020, at https://hdl.handle.net/2027/coo.31924004005975.
California Department of Water Resources, 2004, Coastal Plain of Los Angeles Groundwater Basin, Central Subbasin: California Department of Water Resources, California’s Groundwater Bulletin no. 118, 5 p., accessed March 11, 2022, at https://water.ca.gov/-/media/DWR-Website/Web-Pages/Programs/Groundwater-Management/Bulletin-118/Files/2003-Basin-Descriptions/4_011_04_CentralSubbasin.pdf.
California Department of Water Resources, 2015, California’s groundwater update 2013—A compilation of enhanced content for California water plan update 2013: California Department of Water Resources web page, accessed December 15, 2020, at https://cawaterlibrary.net/wp-content/uploads/2017/05/GWU2013_Ch6_SouthCoast_Final.pdf.
California Department of Water Resources, 2018, Water data library (WDL) station map: California Department of Water Resources web page, accessed August 2018 at https://www.water.ca.gov/waterdatalibrary/waterquality/index.cfm/.
California Department of Water Resources, 2020, California’s groundwater update 2020: California Department of Water Resources web page, accessed March 28, 2022, at https://data.cnra.ca.gov/dataset/calgw_update2020.
California Department of Water Resources, 2021, Land Subsidence Global Positioning System Stations online data: UNAVCO Inc., accessed September 3, 2021, at https://sgma.water.ca.gov/webgis/?appid=SGMADataViewer#landsub.
California Public Utilities Commission, 2001, Southern California Gas Company Montebello Gas Storage Facility decommissioning and sale: California Public Utilities Commission online project documents, accessed January 2020 at https://www.cpuc.ca.gov/Environment/info/mha/montebello/project_docs.htm.
California State Water Resources Control Board, 2017a, GeoTracker electronic deliverable format (EDF) data: California State Water Resources Control Board web page, accessed May 2017 at https://geotracker.waterboards.ca.gov/data_download_by_county.
California State Water Resources Control Board, 2017b, GeoTracker GAMA web page: California State Water Resources Control Board, accessed July 17, 2017, at https://geotracker.waterboards.ca.gov/gama/.
California State Water Resources Control Board, 2019a, Water quality in areas of oil and gas production—Regional Groundwater Monitoring: California State Water Resources Control Board web page, accessed January 7, 2019, at https://www.waterboards.ca.gov/water_issues/programs/groundwater/sb4/regional_monitoring/.
California State Water Resources Control Board, 2019b, GeoTracker map web page: California State Water Resources Control Board web page, accessed September 18, 2019, at https://geotracker.waterboards.ca.gov/map/.
California State Water Resources Control Board, 2019c, California drinking water-related laws: California State Water Resources Control Board web page, accessed March 26, 2020, at https://www.waterboards.ca.gov/drinking_water/certlic/drinkingwater/Lawbook.shtml.
California State Water Resources Control Board Division of Drinking Water, 2018, EDT library and water quality analyses data and download page: California State Water Resources Control Board Division of Drinking Water digital data, accessed June 19, 2018, at https://www.waterboards.ca.gov/drinking_water/certlic/drinkingwater/EDTlibrary.shtml.
California State Water Resources Control Board Division of Drinking Water, 2020, Drinking water notification levels and response levels—An overview: California State Water Resources Control Board Division of Drinking Water, 16 p., accessed December 7, 2020, at https://www.waterboards.ca.gov/drinking_water/certlic/drinkingwater/NotificationLevels.html.
Catalyst Environmental Solutions, 2016, Response to 13267 Investigative Order No. R4-2015-0316 regarding disposal of well and production fluids: Catalyst Environmental Solutions, Technical Memorandum prepared for Freeport-McMoRan Oil and Gas, 28 p., accessed May 24, 2021, at https://www.waterboards.ca.gov/losangeles/water_issues/programs/Oil_and_Gas/Sumps_and_Storage/R4-2015-0316/2016%2002%2001_CES%20Technical%20Report.pdf.
Chernoff, G., Bosan, W., and Oudiz, D., 2008, Determination of a Southern California regional background arsenic concentration in soil: California Department of Toxic Substances Control document, accessed November 15, 2022, at https://www.sandiegocounty.gov/content/dam/sdc/pds/ceqa/Bagley-Major-Grading-Plan-Change/Determination-of-Background-Arsenic.pdf.
Compton, J.S., Williams, L.B., and Ferrell, R.E., Jr., 1992, Mineralization of organogenic ammonium in the Monterey Formation, Santa Maria and San Joaquin basins, California, USA: Geochimica et Cosmochimica Acta, v. 56, no. 5, p. 1979–1991. [Available at https://doi.org/10.1016/0016-7037(92)90324-C.]
County of Los Angeles, 2020, Water conservation facility for retaining stormwater: County of Los Angeles digital data, accessed March 28, 2022, at https://egis-lacounty.hub.arcgis.com/datasets/lacounty::spreading-grounds-feature-layer/about.
Crain, E.R., 2010, Crain’s petrophysical handbook: Mud logging and mud gas logging web page, accessed August 31, 2020, at https://www.spec2000.net/08-mud.htm.
Dale, B.A., Narahara, G.M., and Stevens, R.M., 2000, Case history of reservoir subsidence and wellbore damage management in the South Belridge Diatomite Field: SPE Production & Facilities, v. 15, no. 1, p. 50–57. [Available at https://doi.org/10.2118/60844-PA.]
Darrah, T.H., Vengosh, A., Jackson, R.B., Warner, N.R., and Poreda, R.J., 2014, Noble gases identify the mechanisms of fugitive gas contamination in drinking-water wells overlying the Marcellus and Barnett Shales: Proceedings of the National Academy of Sciences of the United States of America, v. 111, no. 39, p. 14076–14081. [Available at https://doi.org/10.1073/pnas.1322107111.]
Davis, T.A., Landon, M.K., and Bennett, G.L., 2018a, Prioritization of oil and gas fields for regional groundwater monitoring based on a preliminary assessment of petroleum resource development and proximity to California’s groundwater resources: U.S. Geological Survey Scientific Investigations Report 2018–5065, 115 p., accessed December 14, 2020, at https://doi.org/10.3133/sir20185065.
Davis, T.A., Teunis, J.A., McCarlson, A.J., Seitz, N.O., and Johnson, J.C., 2018b, Water chemistry data for samples collected at groundwater and surface-water sites near the Lost Hills and Belridge oil fields, November 2016–September 2017, Kern County, California: U.S. Geological Survey data release, https://doi.org/10.5066/F7NS0T5M.
Davies, R.J., Almond, S., Ward, R.S., Jackson, R.B., Adams, C., Worrall, F., Herringshaw, L.G., Gluyas, J.G., and Whitehead, M.A., 2014, Oil and gas wells and their integrity—Implications for shale and unconventional resource exploitation: Marine and Petroleum Geology, v. 56, p. 239–254, accessed September 20, 2021. [Available at https://doi.org/10.1016/j.marpetgeo.2014.03.001.]
Dawson, B.J.M., Belitz, K., Land, M., and Danskin, W.R., 2003, Stable isotopes and volatile organic compounds along seven ground-water flow paths in divergent and convergent flow systems, southern California, 2000: U.S. Geological Survey Water-Resources Investigations Report 2003–4059, 79 p. [Available at https://doi.org/10.3133/wri034059.]
Dillon, D.B., Davis, T.A., Landon, M.K., Land, M., Wright, M.T., and Kulongoski, J.T., 2016, Data from exploratory sampling of groundwater in selected oil and gas areas of coastal Los Angeles County and Kern and Kings Counties in southern San Joaquin Valley, 2014–15: U.S. Geological Survey Open-File Report 2016–1181, 24 p. [Available at https://doi.org/10.3133/ofr20161181.]
Dusseault, M.B., Gray, M.N., and Nawrocki, P.A., 2000, Why oil wells leak—Cement behavior and long-term consequences: Beijing, China, International Oil and Gas Conference and Exhibition, November 7–10, 2000, 8 p., accessed September 20, 2021, at https://doi.org/10.2118/64733-MS.
Dusseault, M.B., Jackson, R.E., and MacDonald, D., 2014, Towards a road map for mitigating the rates and occurrences of long-term wellbore leakage: Ottawa, Ontario, Canada, University of Waterloo and Geofirma Engineering Ltd., 69 p., accessed September 20, 2021, at https://www.geofirma.com/Links/Wellbore_Leakage_Study%20compressed.pdf.
Edwards, B.D., Hanson, R.T., Reichard, E.G., and Johnson, T.A., 2009, Characteristics of Southern California coastal aquifer systems: Geological Society of America Special Paper, v. 254, p. 319–344, accessed December 15, 2020, at https://doi.org/10.1130/2009.2454(5.2).
Eichhubl, P., and Boles, J.R., 2000, Focused fluid flow along faults in the Monterey Formation, coastal California: Geological Society of America Bulletin, v. 112, no. 11, p. 1667–1679. [Available at https://www.beg.utexas.edu/eichhubl/Reprints/GSABull00.pdf.]
Entrix, Inc., 2009, Remedial action plan—Plains Exploration & Production Company, Montebello Oil Field, Montebello, California: Ventura, Calif., Entrix, Inc., report prepared for Plains Exploration & Production Company, project no. 3086127, 190 p., accessed April 15, 2019, at https://documents.geotracker.waterboards.ca.gov/esi/uploads/geo_report/3602471768/SLT43355353.PDF.
Falcone, J.A., 2015, U.S. conterminous wall-to-wall anthropogenic land use trends (NWALT), 1974–2012: U.S. Geological Survey Data Series 948, 33 p. plus appendixes 3–6 as separate files, accessed January 14, 2020, at https://doi.org/10.3133/ds948.
Fram, M.S., and Belitz, K., 2012, Groundwater quality in the Coastal Los Angeles Basin, California: U.S. Geological Survey Fact Sheet 2012–3096, 4 p. [Available at https://doi.org/10.3133/70039952.]
Gillespie, J.M., Davis, T.A., Stephens, M.J., Ball, L.B., and Landon, M.K., 2019, Groundwater salinity and the effects of produced water disposal in the Lost Hills–Belridge oil fields, Kern County, California—AAPG: Environmental Geoscience, v. 26, no. 3, p. 73–96. [Available at https://doi.org/10.1306/eg.02271918009.]
Goldrath, D., Fram, M.S., Land, M., and Belitz, K., 2012, Status of groundwater quality in the Coastal Los Angeles Basin, 2006—California GAMA Priority Basin Project: U.S. Geological Survey Scientific Investigations Report 2012–5048, 64 p. [Available at https://doi.org/10.3133/sir20125048.]
Hammond, P.A., Wen, T., Brantley, S.L., and Engelder, T., 2020, Gas well integrity and methane migration—Evaluation of published evidence during shale-gas development in the USA: Hydrogeology Journal, v. 28, no. 4, p. 1481–1502, accessed May 25, 2021, at https://doi.org/10.1007/s10040-020-02116-y.
Hodgson, S.F., 1987, Onshore oil and gas seeps in California: California Department of Conservation, Division of Oil and Gas, publication no. TR26, 97 p., accessed March 2020 at https://data.ca.gov/dataset/calgem-tr26-onshore-seeps.
Hornafius, J.S., Quigley, D., and Luyendyk, B.P., 1999, The world’s most spectacular marine hydrocarbon seeps (Coal Oil Point, Santa Barbara Channel, California)—Quantification of emissions: Journal of Geophysical Research, v. 104, no. C9, p. 20703–20711, accessed September 20, 2021, at https://doi.org/10.1029/1999JC900148.
Ingraffea, A.R., Wells, M.T., Santoro, R.L., and Shonkoff, S.B.C., 2014, Assessment and risk analysis of casing and cement impairment in oil and gas wells in Pennsylvania, 2000–2012: Proceedings of the National Academy of Sciences of the United States of America, v. 111, no. 30, p. 10955–10960, accessed September 20, 2021, at https://doi.org/10.1073/pnas.1323422111.
Izbicki, J.A., Borchers, J.W., Leighton, D.A., Kulongoski, J., Fields, L., Galloway, D.L., and Michel, R.L., 2003, Hydrogeology and geochemistry of aquifers underlying the San Lorenzo and San Leandro areas of the East Bay Plain, Alameda County, California: U.S. Geological Survey Water-Resources Investigations Report 2002–4259, 61 p. [Available at https://doi.org/10.3133/wri024259.]
Izbicki, J.A., Christensen, A.H., Newhouse, M.W., and Aiken, G.R., 2005a, Inorganic, isotopic, and organic composition of high-chloride water from wells in a coastal southern California aquifer: Applied Geochemistry, v. 20, no. 8, p. 1496–1517. [Available at https://doi.org/10.1016/j.apgeochem.2005.04.010.]
Izbicki, J.A., Christensen, A.H., Newhouse, M.W., Smith, G.A., and Hanson, R.T., 2005b, Temporal changes in vertical distribution of flow and chloride in deep wells: Groundwater, v. 43, no. 4, p. 531–544. [Available at https://doi.org/10.1111/j.1745-6584.2005.0032.x.]
Jackson, R.E., Gorody, A.W., Mayer, B., Roy, J.W., Ryan, M.C., and Van Stempvoort, D.R., 2013, Groundwater protection and unconventional gas extraction—The critical need for field-based hydrogeological research: Groundwater, v. 51, no. 4, p. 488–510. [Available at https://doi.org/10.1111/gwat.12074.]
Johnson, T.D., and Belitz, K., 2009, Assigning land use to supply wells for the statistical characterization of regional groundwater quality—Correlating urban land use and VOC occurrence: Journal of Hydrology, v. 370, no. 1–4, p. 100–108. [Available at https://doi.org/10.1016/j.jhydrol.2009.02.056.]
de Jong, M., Moran, J.E., and Visser, A., 2020, Identifying paleowater in California drinking water wells: Quaternary International, v. 547, p. 197–207. [Available at https://doi.org/10.1016/j.quaint.2019.04.008.]
Jung, B., Garven, G., and Boles, J.R., 2015, The geodynamics of faults and petroleum migration in the Los Angeles Basin, California: American Journal of Science, v. 315, no. 5, p. 412–459. [Available at https://doi.org/10.2475/05.2015.02.]
Jurgens, B.C., Fram, M.S., Belitz, K., Burow, K.R., and Landon, M.K., 2010, Effects of groundwater development on uranium—Central Valley, California, USA: Groundwater, v. 48, no. 6, p. 913–928. [Available at https://doi.org/10.1111/j.1745-6584.2009.00635.x.]
Karubian, R.Y., 1939, Surface and subsurface geology of Montebello Hills, California: Pasadena, Calif., California Institute of Technology, master’s thesis, 44 p., accessed March 15, 2019, at https://thesis.library.caltech.edu/5568/.
King, G.E., and King, D.E., 2013, Environmental risk arising from well construction failure—Difference between barrier and well failure, and estimates of failure frequency across common well types, locations and well age: New Orleans, La., SPE Annual Technical Conference and Exhibition, September 30–October 2, 2013, p. 323–344, accessed September 20, 2021, at https://doi.org/10.2118/166142-MS.
Kulongoski, J.T., McMahon, P.B., Land, M., Wright, M.T., Johnson, T.A., and Landon, M.K., 2018, Origin of methane and sources of high concentrations in Los Angeles groundwater: Journal of Geophysical Research. Biogeosciences, v. 123, no. 3, p. 818–831. [Available at https://doi.org/10.1002/2017JG004026.]
Land, M., Reichard, E.G., Crawford, S.M., Everett, R.R., Newhouse, M.W., and Williams, C.F., 2004, Ground-water quality of coastal aquifer systems in the West Coast Basin, Los Angeles County, California, 1999–2002: U.S. Geological Survey Scientific Investigations Report 2004–5067, 80 p. [Available at https://doi.org/10.3133/sir20045067.]
Lawrence, S.J., 2006, Description, properties, and degradation of selected volatile organic compounds detected in ground water—A review of selected literature: U.S. Geological Survey Open-File Report 2006–1338, 62 p. [Available at https://doi.org/10.3133/ofr20061338.]
Los Angeles County Sanitation Districts, 2021, 31st annual status report on recycled water use FY 2019–20: Los Angeles County Sanitation Districts online report, accessed July 6, 2021, at https://www.lacsd.org/home/showpublisheddocument/2442/637643175461970000.
Lucas, L.L., and Unterweger, M.P., 2000, Comprehensive review and critical evaluation of the half-life of tritium: Journal of Research of the National Institute of Standards and Technology, v. 105, no. 4, p. 541–549. [Available at https://doi.org/10.6028/jres.105.043.]
McMahon, P.B., Kulongoski, J.T., Wright, M.T., Land, M., Landon, M.K., Cozzarelli, I.M., Vengosh, A., and Aiken, G.R., 2017, Preliminary results from exploratory sampling of wells for the California oil, gas, and groundwater program, 2014–15 (ver. 1.1, January 2017): U.S. Geological Survey Open-File Report 2016–1100, 8 p. [Available at https://doi.org/10.3133/ofr20161100.]
McMahon, P.B., Kulongoski, J.T., Vengosh, A., Cozzarelli, I.M., Landon, M.K., Kharaka, Y.K., Gillespie, J.M., and Davis, T.A., 2018, Regional patterns in the geochemistry of oil-field water, southern San Joaquin Valley, California, USA: Applied Geochemistry, v. 98, p. 127–140. [Available at https://doi.org/10.1016/j.apgeochem.2018.09.015.]
Metzger, L.F., Morris, D., and Watson, E., 2020, Historical produced water chemistry data compiled for selected oil fields in Los Angeles and Orange Counties, southern California: U.S. Geological Survey data release, accessed March 30, 2020, at https://doi.org/10.5066/P9JXQ3SD.
Myer, L., Jacobsen, J., Horsman, J., Fredrich, J.T., Wawersik, W.R., Arguello, J.G., Bruno, M., and Qian, H., 1996, Use of visualization techniques in analysis of well failures in diatomite reservoirs: The Leading Edge, v. 15, no. 3, 5 p. [Available at https://doi.org/10.1190/1.1437290.]
Orem, W.H., Tatu, C., Varonka, M., Lerch, H., Bates, A., Engle, M., Crosby, L., and McIntosh, J., 2014, Organic substances in produced and formation water from unconventional natural gas extraction in coal and shale: International Journal of Coal Geology, v. 126, p. 20–31. [Available at https://doi.org/10.1016/j.coal.2014.01.003.]
Paulinski, S., ed., 2021, Development of a groundwater-simulation model in the Los Angeles Coastal Plain, Los Angeles County, California: U.S. Geological Survey Scientific Investigations Report 2021–5088, 489 p. [Available at https://doi.org/10.3133/sir20215088.]
Piper, A.M., 1944, A graphic procedure in the geochemical interpretations of water analyses: Transactions American Geophysical Union, v. 25, no. 6, p. 914–928. [Available at https://doi.org/10.1029/TR025i006p00914.]
Piper, A.M., and Garrett, A.A., 1953, Native and contaminated ground waters in the Long Beach-Santa Ana area, California: U.S. Geological Survey Water-Supply Paper 1136, 320 p. [Available at https://pubs.er.usgs.gov/publication/wsp1136.]
Plummer, N.L., and Sprinkle, C.L., 2001, Radiocarbon dating of dissolved inorganic carbon in groundwater from confined parts of the Upper Floridan aquifer, Florida, USA: Hydrogeology Journal, v. 9, p. 127–150. [Available at https://doi.org/10.1007/s100400000121.]
Ponti, D.J., Wagner, B.J., Land, M., and Landon, M.K., 2014, Characterization of potential transport pathways and implications for groundwater management near an anticline in the Central Basin area, Los Angeles County, California: U.S. Geological Survey Open-File Report 2014–1087, 75 p. and appendix. [Available at https://doi.org/10.3133/ofr20141087.]
Quarles, M.W., 1941, Geology of the Repetto and Montebello Hills: Pasadena, Calif., California Institute of Technology, master's thesis, 77 p., accessed March 15, 2019, at https://thesis.library.caltech.edu/5586/.
Reichard, E.G., Land, M., Crawford, S.M., Johnson, T., Everett, R.R., Vulshan, T.V., Ponti, D.J., Halford, K.L., Johnson, T.A., Paybins, K.S., and Nishikawa, T., 2003, Geohydrology, geochemistry, and ground-water simulation-optimization of the Central and West Coast Basins, Los Angeles County, California: U.S. Geological Survey Water-Resources Investigations Report 2003–4065, 184 p., accessed December 15, 2020, at https://doi.org/10.3133/wri034065.
Richter, B.C., and Kreitler, C.W., 1991, Identification of sources of groundwater salinization using geochemical techniques: Ada, Okla., U.S. Environmental Protection Agency EPA/600/2-91/064, 272 p., accessed December 14, 2020, at https://nepis.epa.gov.
Rodriguez, O., Stanton. J.S., Marcusa, J.A., Davis, T.A., Johnson, J.C., McCarlson, A.J., and Qi, S.L., 2022, Water chemistry data for samples collected at groundwater sites in the Montebello Oil Field study area, September 2014–October 2018, Los Angeles County, California: U.S. Geological Survey data release, https://doi.org/10.5066/P9FZ2SBH.
Rosecrans, C.Z., Landon, M.K., McMahon, P.B., Gillespie, J.M., Kulongoski, J.T., Stephens, M.J., Hunt, A.G., Shimabukuro, D.H., and Davis, T.A., 2021, Groundwater quality of aquifers overlying the Oxnard Oil Field, Ventura County, California: Science of the Total Environment, v. 771, p. 144822. [Available at https://doi.org/10.1016/j.scitotenv.2020.144822.]
Saucedo, G.J., Bedford, D.R., Raines, G.L., Miller, R.J., Wentworth, C.M., Jennings, C.W., Strand, R.G., and Rogers, T.H., 2000, GIS data for the geologic map of California: California Department of Conservation, California Geological Survey, accessed January 20, 2021, at https://ngmdb.usgs.gov/Prodesc/proddesc_43655.htm.
Save The Montebello Hills Task Force, 2011, The cultural impact of the Montebello Hills oil field: Save The Montebello Hills Task Force, The Montebello Hills Oil Field Fact Sheet 1, accessed March 15, 2019, at https://angeles.sierraclub.org/save_montebello_hills_task_force.
Schoell, M., 1980, The hydrogen and carbon isotopic composition of methane from natural gases of various origins: Geochimica et Cosmochimica Acta, v. 44, no. 5, p. 649–661. [Available at https://doi.org/10.1016/0016-7037(80)90155-6.]
Shelton, J.L., Burow, K.R., Belitz, K., Dubrovsky, N.M., Land, M., and Gronberg, J., 2001, Low-level volatile organic compounds in active public supply wells as ground-water tracers in the Los Angeles physiographic basin, California, 2000: U.S. Geological Survey Water-Resources Investigations Report 2001–4188, 35 p., accessed December 15, 2020, at https://pubs.usgs.gov/wri/2001/wri014188/.
Shimabukuro, D.H., Stephens, M.J., Crandall-Bear, A., Gillespie, J.M., Haugen, E.A., and Chang, W., 2019, Mapping volume balance of injected and produced fluids in and around Kern County oil fields—American Association of Petroleum Geologists Pacific Section Convention, 2019, Long Beach, California, April 1–3, 2019: Online abstract, accessed July 18, 2022, at https://www.searchanddiscovery.com/abstracts/html/2019/longbeach-90339/abstracts/2019.PS.04.html.
Swanson, R.G., 1981, Sample examination manual: American Association of Petroleum Geologists methods in exploration: American Association of Petroleum Geologists, no. 1, 54 p., accessed December 16, 2020, at https://doi.org/10.1306/Mth1413.
Swanson, G.S., 2018, Injection well rate and pressure analyses suggest practical waterflood improvements: Garden Grove, Calif., Society of Petroleum Engineers Western Regional Meeting proceedings, April 22–27, 2018, accessed September 2, 2021, at https://doi.org/10.2118/190030-MS.
Taylor, S.W., Sherwood Lollar, B., and Wassenaar, I., 2000, Bacteriogenic ethane in near-surface aquifers—Implications for leaking hydrocarbon well bores: Environmental Science & Technology, v. 34, no. 22, p. 4727–4732. [Available at https://doi.org/10.1021/es001066x.]
Tyne, R.L., Barry, P.H., Karolyte, R., Byrne, D.J., Kulongoski, J.T., Hillegonds, D.J., and Ballentine, C.J., 2021, Investigating the effect of enhanced oil recovery on the noble gas signature of casing gases and produced waters from selected California oil fields: Chemical Geology, v. 584, 10 p., accessed December 29, 2021, at https://doi.org/10.1016/j.chemgeo.2021.120540.
U.S. Energy Information Administration, 2020, California state profile and energy estimates: U.S. Energy Information Administration web page, accessed October 6, 2020, at https://www.eia.gov/state/seds/seds-data-complete.php?sid=CA#stateSelection.
U.S. Environmental Protection Agency, 2006, First five-year review report for San Gabriel Valley area 1 Superfund Site, Whittier Narrows Operable Unit, Los Angeles County, California: U.S. Environmental Protection Agency report, 84 p., accessed March 13, 2020, at https://semspub.epa.gov/work/09/1140256.pdf.
U.S. Environmental Protection Agency, 2011, Second five-year review report for San Gabriel Valley area 1 Superfund site, Whittier Narrows Operable Unit, Los Angeles County, California: U.S. Environmental Protection Agency report, 146 p., accessed March 13, 2020, at https://semspub.epa.gov/work/09/2243227.pdf.
U.S. Environmental Protection Agency, 2015, Fifth five-year review report for Operating Industries, Inc. landfill Superfund site, Los Angeles County, California: U.S. Environmental Protection Agency report, 109 p., accessed December 2, 2020, at https://semspub.epa.gov/work/09/1155733.pdf.
U.S. Environmental Protection Agency, 2016, Hydraulic fracturing for oil and gas—Impacts from the hydraulic fracturing water cycle on drinking water resources in the United States: U.S. Environmental Protection Agency report EPA-600-R-16-236Fa, 666 p., accessed September 1, 2021, at https://www.epa.gov/hfstudy.
U.S. Environmental Protection Agency, 2017, National Priorities List (NPL) site polygons, Region 9: U.S. Environmental Protection Agency digital data, accessed March 17, 2020, at https://edg.epa.gov/data/PUBLIC/R9/NationalPriorityListPoint_R9_2019_R9.gdb.zip.
U.S. Environmental Protection Agency, 2019, Aquifer exemptions in the underground injection control program: U.S. Environmental Protection Agency web page, accessed December 2019 at https://www.epa.gov/uic/aquifer-exemptions-underground-injection-control-program.
U.S. Environmental Protection Agency, 2020a, Toxics Release Inventory Program basic data files—Calendar years 1987–present: U.S. Environmental Protection Agency web page, accessed June 18, 2020, at https://www.epa.gov/toxics-release-inventory-tri-program/tri-basic-data-files-calendar-years-1987-2018.
U.S. Environmental Protection Agency, 2020b, Sixth five-year review report for Operating Industries, Inc. Landfill Superfund Site, Los Angeles County, California: U.S. Environmental Protection Agency report, 72 p., accessed December 21, 2020, at https://cumulis.epa.gov/supercpad/SiteProfiles/index.cfm?fuseaction=second.scs&id=0902673&doc=Y&colid=39481®ion=09&type=SC.
U.S. Geological Survey, 1998, Water quality samples for California—USGS 335013118142501: U.S. Geological Survey National Water Information System, accessed July 13, 2021, at https://nwis.waterdata.usgs.gov/ca/nwis/qwdata/?site_no=335013118142501.
U.S. Geological Survey, 2015, Changes to National Water Quality Laboratory procedures used to establish and verify laboratory detection and reporting limits: U.S. Geological Survey National Water Quality Laboratory Technical Memorandum 15.02, accessed September 16, 2021, at https://nwql.usgs.gov/tech_memos/nwql.2015-02.pdf.
U.S. Geological Survey, 2018, Health-based screening levels for evaluating water-quality data: U.S. Geological Survey web page, accessed on December 7, 2020, at https://water.usgs.gov/water-resources/hbsl/.
U.S. Geological Survey, 2019, California Oil, Gas, and Groundwater (COGG) Program web page: U.S. Geological Survey, accessed April 7, 2019, at https://ca.water.usgs.gov/projects/oil-gas-groundwater/.
U.S. Geological Survey, 2021, USGS water data for the Nation: U.S. Geological Survey National Water Information System database, accessed January 12, 2021, at https://doi.org/10.5066/F7P55KJN.
U.S. Geological Survey, variously dated, The national field manual for the collection of water-quality data (ver. 7): U.S. Geological Survey Techniques and Method, book 9, chaps. A1–A9, accessed April 5, 2019, at https://water.usgs.gov/owq/FieldManual/.
United Water Conservation District, 2021, Saline intrusion and 2020 groundwater conditions update, Oxnard and Pleasant Valley basins: United Water Conservation District Open-File Report 2021–03, 108 p., accessed March 23, 2022, at https://www.unitedwater.org/wp-content/uploads/2021/12/UWCD-OFR-2021-03-Saline-Intrusion-and-2020-GW-Conditions-Update-Oxnard-and-PV-Basins.pdf.
URS Corporation, 2015, Performance evaluation report for 2014—San Gabriel Valley Area 1 Superfund Site, Whitter Narrows Operable Unit: URS Project no. 29403647, 144 p., accessed January 29, 2019, at https://www.envirostor.dtsc.ca.gov/public/final_documents2?global_id=60001340&doc_id=60360502.
Vengosh, A., Jackson, R.B., Warner, N., Darrah, T.H., and Kondash, A., 2014, A critical review of the risks to water resources from unconventional shale gas development and hydraulic fracturing in the United States: Environmental Science & Technology, v. 48, no. 15, p. 8334–8348. [Available at https://doi.org/10.1021/es405118y.]
Water Replenishment District of Southern California, 2016, Regional groundwater monitoring report water year 2014–2015: Lakewood, Calif., Water Replenishment District of Southern California, 193 p., accessed August 25, 2020, at https://www.wrd.org/files/0d078c533/Regional+Groundwater+Monitoring+Report+2014-2015.pdf.
Water Replenishment District of Southern California, 2019a, Engineering survey and report, 2019: Lakewood, Calif., Water Replenishment District of Southern California Report, 76 p., accessed August 28, 2020, at https://www.wrd.org/files/109c06156/2019+Engineering+Survey+and+Report.pdf.
Water Replenishment District of Southern California, 2019b, Well search tool: Water Replenishment District of Southern California web page, accessed May 2019 at https://gis.wrd.org/.
Water Replenishment District of Southern California, 2020, The Water Independence Now Program: Water Replenishment District of Southern California web page, accessed April 16, 2020, at https://www.wrd.org/water-independence-now-win.
Water Replenishment District of Southern California, variously dated, Regional groundwater monitoring report: Lakewood, Calif., Water Replenishment District of Southern California web page, accessed May 16, 2019, at https://www.wrd.org/regional-groundwater-monitoring-report.
Watson, T.L., and Bachu, S., 2009, Evaluation of the potential for gas and CO2 leakage along wellbores: SPE Drilling & Completion, v. 24, no. 1, p. 115–126. [Available at https://doi.org/10.2118/106817-PA.]
Whiticar, M.J., Faber, E., and Schoell, M., 1986, Biogenic methane formation in marine and freshwater environments—CO2 reduction vs. acetate fermentation—Isotopic evidence: Geochimica et Cosmochimica Acta, v. 50, no. 5, p. 693–709. [Available at https://doi.org/10.1016/0016-7037(86)90346-7.]
Wisen, J., Chesnaux, R., Werring, J., Wendling, G., Baudron, P., and Barbecot, F., 2020, A portrait of wellbore leakage in northeastern British Columbia, Canada: Proceedings of the National Academy of Sciences of the United States of America, v. 117, no. 2, p. 913–922. [Available at https://doi.org/10.1073/pnas.1817929116.]
Wright, T.L., 1991, Structural geology and tectonic evolution of the Los Angeles Basin, California, chap. 3 in Biddle, K.T., ed., Active margin basins: Tulsa, Okla., American Association of Petroleum Geologists Memoir 52, p. 35–134. [Available at https://doi.org/10.1306/M52531C3.]
Wright, M.T., McMahon, P.B., Landon, M.T., and Kulongoski, J.T., 2019, Groundwater quality of a public supply aquifer in proximity to oil development, Fruitvale oil field, Bakersfield, California: Applied Geochemistry, v. 106, p. 82–95. [Available at https://doi.org/10.1016/j.apgeochem.2019.05.003.]
Appendix 1. Glossary
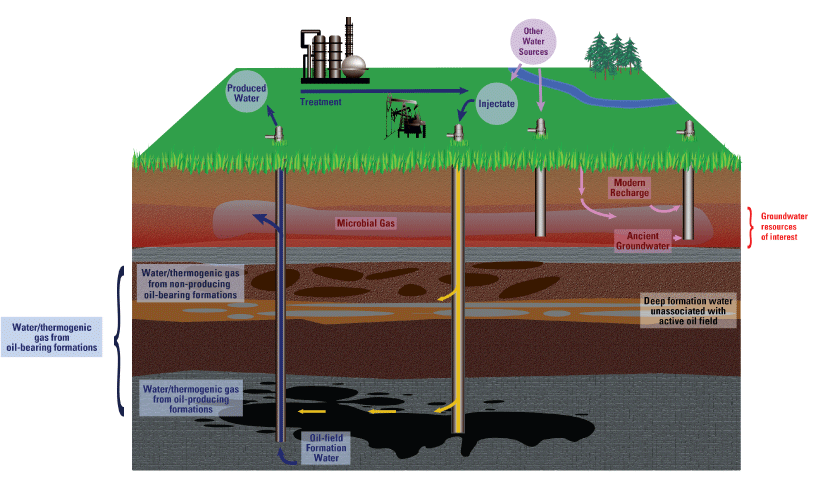
Conceptual diagram illustrating oil-field features and sources of water and petroleum hydrocarbons.
Terms Related to Sources
-
Oil and gas sources of chemical constituents: include oil- and gas-development activities and naturally occurring (geologic) oil, gas, and saline water in sediments near oil fields. Synonyms: oil-production activities, oil-bearing formations, hydrocarbon-bearing formations.
-
Naturally occurring sources of hydrocarbons: naturally occurring oil and gas shows in sediments overlying or near oil fields; these do not yield commercial quantities of oil or gas.
Terms Related to Water
-
Water from oil-bearing formations: water having elevated salinity and concentrations of inorganic or organic solutes derived from oil- and gas-bearing formations, including those used for commercial oil and gas production and naturally occurring oil and gas shows in overlying sediments.
-
Water from oil-producing formations: water derived from an oil-field source. This source can include produced water, oil-field formation water, and injectate (water intended for disposal or injection for secondary recovery). Differs from produced water in terms of how the sample is accessed. This source would not include water potentially derived from naturally hydrocarbon-bearing sediments overlying oil fields that do not yield commercial quantities of oil or gas.
-
Produced water: water withdrawn from an oil well and brought to the surface and may include oil, water, and gas from the geologic formation and water or gas injected for enhanced recovery.
-
Oil-field formation water: water of original composition in the pore spaces of oil-producing formations that has not been altered by injection processes.
-
Injectate: water injected for disposal or for secondary recovery. Generally, consists primarily of recycled produced water, with treatments such as oil skimming (dehydrogenation), sometimes with treatment or additives to limit biological activity, corrosion, and buildup of mineral deposits in injection wells. In some fields, injection for disposal is outside of oil-producing zones.
-
Water from non-producing oil-bearing formations: water from sediments containing naturally occurring oil and gas shows that overlie oil-producing zones.
-
Deep formation water unassociated with active oil fields: water having elevated salinity (concentrations of inorganic constituents and other dissolved solutes) derived from relatively deep formations not associated with oil and gas fields.
-
Idle well-fluid level: a fluid level measured in a well that has not produced oil or gas, produced water to be used in production stimulation, or been used for enhanced oil recovery, reservoir pressure management, or injection for a period of 24 consecutive months.
Terms Related to Gas
-
Thermogenic gas from oil-bearing formations: hydrocarbon gas of thermogenic origin derived from oil- and gas-bearing formations, including those used for commercial oil and gas production and naturally occurring oil and gas shows in overlying sediments.
-
Thermogenic gas from oil-producing formations: thermogenic gas derived from an oil-field source. This source can include produced water, oil-field formation water, and injectate (water intended for disposal or injection for secondary recovery). This would not include water potentially derived from naturally hydrocarbon-bearing sediments overlying oil fields that do not yield commercial quantities of oil or gas.
-
Thermogenic gas from non-producing oil-bearing formations: thermogenic gas from sediments containing naturally occurring oil and gas shows that overlie oil-producing zones.
-
Thermogenic gas: natural gas, including methane and heavier light hydrocarbon gases derived from oil and gas generation processes that include heating and high pressure, typically originally occurring at relatively large depths.
-
Microbial gas: natural gas, generally including methane with sometimes small amounts of ethane, derived from in situ production by microbe-facilitated reactions with organic carbon.
Appendix 2. Data Quality
Table 2.1.
Quality-control samples used for evaluating data quality of Regional Monitoring Program groundwater samples collected for the Montebello Oil Field study area (2018).References Cited
Dillon, D.B., Davis, T.A., Landon, M.K., Land, M.T., Wright, M.T., and Kulongoski, J.T., 2016, Data from exploratory sampling of groundwater in selected oil and gas areas of coastal Los Angeles County and Kern and Kings Counties in southern San Joaquin Valley, 2014–15—California oil, gas, and groundwater project: U.S. Geological Survey Open-File Report 2016–1181, 24 p. [Available at https://doi.org/10.3133/ofr20161181.]
Rodriguez, O., Stanton. J.S., Marcusa, J.A., Davis, T.A., Johnson, J.C., McCarlson, A.J., and Qi, S.L., 2022, Water chemistry data for samples collected at groundwater sites in the Montebello Oil Field study area, September 2014–October 2018, Los Angeles County, California: U.S. Geological Survey data release, https://doi.org/10.5066/P9FZ2SBH.
Appendix 3. Supplemental Tables
Table 3.1.
Well-integrity issues and base of freshwater noted in reports for oil and gas wells within 500 meters of a Regional Monitoring Program groundwater sample in the Montebello Oil Field study area (California Department of Conservation, 2020c).[API, American Petroleum Institute; m, meter; BFW, base of freshwater; OG, oil and gas well; GS, gas storage well; OB, observation well. Types of well-integrity issues: Casing damage; casing holes or leaks, parted or collapsed casing; open hole, extensive intervals of hole are without casings and cement plugs; blow out, unintended flow of oil, gas, and water to surface]
Number of oil wells does not match total count in table 2 because five oil wells were within 500 m of more than one Regional Monitoring Program sample.
Depth to base of underground source of drinking water (USDW; U.S. Environmental Protection Agency, 2019) was noted in well reports as 632 m for 0403721918, 0403700054, 0403700055, 0403712586, 0403712769, 0403712771, 0403712774, and 0403721972; 627 m for 0403700057, 0403700550, and 0403700631; and 1,137 m for 0403700819.
Table 3.2.
Oil and gas shows in mud logs in the Montebello Oil Field study area (California Department of Conservation, 2019b).[API, American Petroleum Institute; m, meter; C3–C5, propane through pentane; OG, oil and gas well; P, plugged and abandoned; —, no data available]
Table 3.3.
Depth to idle oil well fluid levels and hydrocarbons within 500 meters of Regional Monitoring Program groundwater sample BNE-3 (California Department of Conservation, 2019b; California Department of Conservation, 2020c)136.[API, American Petroleum Institute; m, meter; NAVD 88, North American Vertical Datum of 1988; OG, oil and gas well; P, plugged and abandoned; —, no data]
Table 3.4.
Volatile organic compounds detected in Regional Monitoring Program groundwater samples in the Montebello Oil Field study area, 2014 and 2017–18 (U.S. Geological Survey, 2021).[VOC, volatile organic compound; µg/L, microgram per liter; MCL-US, maximum contaminant level (U.S. Environmental Protection Agency, 2018); MCL-CA, maximum contaminant level (California State Water Resources Control Board, 2019c); HBSL-NC, non-enforceable U.S. Geological Survey noncancer health-based screening level (U.S. Geological Survey, 2018); RL-CA, California drinking-water response level (California State Water Resources Control Board Division of Drinking Water, 2020); E, estimated]
Categorized as organic synthesis by DeSimone and others (2014) but also could be naturally occurring.
Table 3.5.
Volatile organic compound concentrations and age-dating results for Regional Monitoring Program groundwater samples in the Montebello Oil Field study area, 2014 and 2017–18.[—Left][U.S. Geological Survey, 2021. Abbreviations: ND, not detected above laboratory detection level; VOC, volatile organic compound; µg/L, microgram per liter; E, estimated]
Result type | Sample identifier (fig. 1) | ||||||||
---|---|---|---|---|---|---|---|---|---|
MTE-1 | MTE-2 | BNE-2 | BNE-3 | MT-1 | MT-2 | MT-3 | MT-4 | MT-5 | |
Tritium concentration, in tritium units | ND | 8.84 | 8.09 | ND | 5.83 | 5.74 | 2.76 | 6.55 | 8.19 |
Carbon-14 in dissolved inorganic carbon, in percent modern carbon | 0.13 | 133.56 | 102.17 | 2.64 | 94.43 | 95.95 | 92.57 | 96.40 | 92.76 |
Groundwater age category based on tritium and carbon-14 results (fig. 14) | Pre-modern | Modern | Modern | Pre-modern | Modern | Modern | Modern | Modern | Modern |
Number of manufactured VOCs detected | 0 | 2 | 5 | 0 | 2 | 6 | 3 | 2 | 2 |
Number of petroleum hydrocarbons detected | 0 | 0 | 0 | 1 | 0 | 0 | 0 | 0 | 1 |
Manufactured VOC concentration | |||||||||
Tetrachloroethene (PCE), in µg/L | ND | ND | ND | ND | 0.0444 | 1.51 | ND | 0.0347 | 0.0345 |
Trichloroethene (TCE), in µg/L | ND | ND | 0.0784 | ND | 0.023 | 0.196 | ND | 0.029 | ND |
Trichloromethane (chloroform), in µg/L | ND | ND | ND | ND | ND | 0.0554 | ND | ND | 0.0227 |
cis-1,2-dichloroethene, in µg/L | ND | 0.0296 | 0.192 | ND | ND | 0.062 | ND | ND | ND |
1,1-dichloroethene, in µg/L | ND | ND | ND | ND | ND | 0.020 | ND | ND | ND |
Diethyl ether, in µg/L | ND | 0.084 | 0.077 | ND | ND | ND | 0.043 | ND | ND |
1,1-dichloroethane, in µg/L | ND | ND | 0.0267 | ND | ND | ND | ND | ND | ND |
Methyl tert-butyl ether (MTBE), in µg/L | ND | ND | ND | ND | ND | 0.089 | 0.051 | ND | ND |
Tetrachloromethane (carbon tetrachloride), in µg/L | ND | ND | ND | ND | ND | ND | ND | ND | ND |
trans-1,2-dichloroethene, in µg/L | ND | ND | 0.0259 | ND | ND | ND | ND | ND | ND |
Chlorobenzene, in µg/L | ND | ND | ND | ND | ND | ND | 0.0127 | ND | ND |
Chloroethane, in µg/L | ND | ND | ND | ND | ND | ND | ND | ND | ND |
Trichlorofluoromethane (CFC-11), in µg/L | ND | ND | ND | ND | ND | ND | ND | ND | ND |
1,2-dichloropropane, in µg/L | ND | ND | ND | ND | ND | ND | ND | ND | ND |
1,4-dichlorobenzene, in µg/L | ND | ND | ND | ND | ND | ND | ND | ND | ND |
Dichlorodifluoromethane (CFC-12), in µg/L | ND | ND | ND | ND | ND | ND | ND | ND | ND |
Vinyl chloride, in µg/L | ND | ND | ND | ND | ND | ND | ND | ND | ND |
Petroleum hydrocarbon VOC concentration | |||||||||
Benzene, in µg/L | ND | ND | ND | 0.0150 | ND | ND | ND | ND | 0.0203 |
Ethylbenzene, in µg/L | ND | ND | ND | ND | ND | ND | ND | ND | ND |
o-Xylene, in µg/L | ND | ND | ND | ND | ND | ND | ND | ND | ND |
1,2,4-trimethylbenzene, in µg/L | ND | ND | ND | ND | ND | ND | ND | ND | ND |
m-Xylene plus p-xylene, in µg/L | ND | ND | ND | ND | ND | ND | ND | ND | ND |
4-isopropyltoluene, in µg/L | ND | ND | ND | ND | ND | ND | ND | ND | ND |
Carbon disulfide concentration, in µg/L | E0.076 | ND | ND | E0.044 | ND | ND | ND | ND | ND |
Table 3.5.
Volatile organic compound concentrations and age-dating results for Regional Monitoring Program groundwater samples in the Montebello Oil Field study area, 2014 and 2017–18.[—Right][U.S. Geological Survey, 2021. Abbreviations: VOC, volatile organic compound; µg/L, microgram per liter; ND, not detected above laboratory detection level; E, estimated]
Sample identifier (fig. 1) | |||||||||||
---|---|---|---|---|---|---|---|---|---|---|---|
MT-6 | MT-7 | MT-8 | MT-9 | MT-10 | MT-11 | MT-12 | MT-13 | MT-14 | MT-15 | MT-16 | MT-17 |
0.18 | 4.43 | 5.12 | 0.25 | 3.65 | 1.49 | 3.46 | 4.03 | ND | ND | ND | 0.92 |
0.58 | 93.44 | 90.53 | 24.26 | 86.94 | 73.49 | 109.37 | 102.12 | 38.12 | 38.94 | 107.88 | 88.54 |
Pre-modern | Modern | Modern | Mixed | Mixed | Mixed | Modern | Modern | Pre-modern | Pre-modern | Mixed | Mixed |
0 | 3 | 0 | 1 | 8 | 2 | 10 | 3 | 0 | 0 | 12 | 2 |
0 | 0 | 0 | 1 | 0 | 0 | 0 | 0 | 0 | 2 | 5 | 4 |
Manufactured VOC concentration | |||||||||||
ND | 0.737 | ND | 0.0186 | 0.726 | 0.0444 | 3.07 | 0.0141 | ND | ND | 5.50 | 0.0534 |
ND | ND | ND | ND | 0.779 | ND | 6.30 | 0.020 | ND | ND | 19.6 | 0.021 |
ND | 0.189 | ND | ND | 0.0708 | 0.0949 | 0.278 | ND | ND | ND | 0.0901 | ND |
ND | ND | ND | ND | 0.050 | ND | 0.065 | ND | ND | ND | 10.0 | ND |
ND | 0.082 | ND | ND | 0.276 | ND | 0.943 | ND | ND | ND | 0.068 | ND |
ND | ND | ND | ND | 0.044 | ND | 0.039 | ND | ND | ND | ND | ND |
ND | ND | ND | ND | 0.0375 | ND | 0.0985 | ND | ND | ND | 0.302 | ND |
ND | ND | ND | ND | ND | ND | 0.100 | 0.094 | ND | ND | ND | ND |
ND | ND | ND | ND | 0.0232 | ND | 0.196 | ND | ND | ND | ND | ND |
ND | ND | ND | ND | ND | ND | ND | ND | ND | ND | 0.298 | ND |
ND | ND | ND | ND | ND | ND | ND | ND | ND | ND | ND | ND |
ND | ND | ND | ND | ND | ND | ND | ND | ND | ND | 0.060 | ND |
ND | ND | ND | ND | ND | ND | 0.027 | ND | ND | ND | ND | ND |
ND | ND | ND | ND | ND | ND | ND | ND | ND | ND | 0.260 | ND |
ND | ND | ND | ND | ND | ND | ND | ND | ND | ND | 0.0555 | ND |
ND | ND | ND | ND | ND | ND | ND | ND | ND | ND | E0.028 | ND |
ND | ND | ND | ND | ND | ND | ND | ND | ND | ND | 0.357 | ND |
Petroleum hydrocarbon VOC concentration | |||||||||||
ND | ND | ND | 0.0241 | ND | ND | ND | ND | ND | ND | 0.0693 | 0.0427 |
ND | ND | ND | ND | ND | ND | ND | ND | ND | ND | 0.0354 | 0.0292 |
ND | ND | ND | ND | ND | ND | ND | ND | ND | ND | 0.0437 | 0.0193 |
ND | ND | ND | ND | ND | ND | ND | ND | ND | 0.0186 | 0.0742 | ND |
ND | ND | ND | ND | ND | ND | ND | ND | ND | ND | 0.084 | 0.042 |
ND | ND | ND | ND | ND | ND | ND | ND | ND | 0.022 | ND | ND |
ND | ND | ND | ND | ND | ND | ND | ND | ND | ND | E0.060 | E0.020 |
Table 3.6.
Dissolved gases and carbon isotopes of methane for Regional Monitoring Program groundwater samples in the Montebello Oil Field study area, 2014 and 2017–18.[—Left][U.S. Geological Survey, 2021. Abbreviations: <, less than; nc, not calculated; na, not analyzed because methane concentration was too small; VPDB, Vienna Pee Dee Belemnite; VSMOW, Vienna Standard Mean Ocean Water]
Category | Sample identifier (fig. 1) | ||||||||
---|---|---|---|---|---|---|---|---|---|
MTE-1 | MTE-2 | BNE-2 | BNE-3 | MT-1 | MT-2 | MT-3 | MT-4 | MT-5 | |
Light hydrocarbon gas composition, in mole percent of dissolved gases | |||||||||
Methane (CH4) | 86.51 | 1.21 | 0.5060 | 94.48 | 0.0077 | <0.0001 | 0.2210 | <0.0001 | 0.1540 |
Ethane (C2H6) | 0.0115 | 0.0005 | <0.0001 | 0.0316 | <0.0001 | <0.0001 | 0.0009 | <0.0001 | <0.0001 |
Ethene (C2H4) | <0.0001 | <0.0001 | <0.0001 | <0.0001 | <0.0001 | <0.0001 | <0.0001 | <0.0001 | <0.0001 |
Propane (C3H8) | <0.0001 | <0.0001 | <0.0001 | <0.0001 | <0.0001 | <0.0001 | <0.0001 | <0.0001 | <0.0001 |
Propene (C3H6) | <0.0001 | <0.0001 | <0.0001 | <0.0001 | <0.0001 | <0.0001 | <0.0001 | <0.0001 | <0.0001 |
Isobutane (2-Methylpropane; iC4H10) | <0.0001 | <0.0001 | <0.0001 | <0.0001 | <0.0001 | <0.0001 | <0.0001 | <0.0001 | <0.0001 |
n-Butane (nC4H10) | <0.0001 | <0.0001 | <0.0001 | <0.0001 | <0.0001 | <0.0001 | <0.0001 | <0.0001 | <0.0001 |
Isopentane (2-Methylbutane; iC5H12) | <0.0001 | <0.0001 | <0.0001 | <0.0001 | <0.0001 | <0.0001 | <0.0001 | <0.0001 | <0.0001 |
n-Pentane (nC5H12) | <0.0001 | <0.0001 | <0.0001 | <0.0001 | <0.0001 | <0.0001 | <0.0001 | <0.0001 | <0.0001 |
Higher-molecular-weight hydrocarbons | <0.0001 | <0.0001 | <0.0001 | <0.0001 | <0.0001 | <0.0001 | <0.0001 | <0.0001 | <0.0001 |
Light hydrocarbon gas concentration, in milligrams per liter | |||||||||
Methane (CH4) | 67 | 0.2500 | 0.1200 | 110 | 0.0016 | <0.0002 | 0.0430 | <0.0002 | 0.0320 |
Ethane (C2H6) | 0.0180 | 0.0002 | <0.0002 | 0.0710 | <0.0002 | <0.0002 | 0.0004 | <0.0002 | <0.0002 |
Propane (C3H8) | <0.0004 | <0.0003 | <0.0003 | <0.0003 | <0.0002 | <0.0002 | <0.0002 | <0.0002 | <0.0002 |
Other light hydrocarbon gas properties | |||||||||
Methane/ (ethane+propane), in mole ratio (fig. 13A) | 7,523 | 2,420 | nc | 2,990 | nc | nc | 246 | nc | nc |
Carbon isotopes in methane (δ13C-CH4), in per mil relative to VPDB (figs. 13A, B) | −77.17 | −70.70 | na | −74.70 | na | na | na | na | na |
Hydrogen isotopes in methane (δ2H-CH4), in per mil relative to VSMOW (fig. 13B) | −227.2 | −174.1 | na | −208.7 | na | na | na | na | na |
Table 3.6.
Dissolved gases and carbon isotopes of methane for Regional Monitoring Program groundwater samples in the Montebello Oil Field study area, 2014 and 2017–18.[—Right][U.S. Geological Survey, 2021. Abbreviations: <, less than; na, not analyzed because methane concentration was too small; nc, not calculated; VPDB, Vienna Pee Dee Belemnite; VSMOW, Vienna Standard Mean Ocean Water]
Sample identifier (fig. 1) | |||||||||||
---|---|---|---|---|---|---|---|---|---|---|---|
MT-6 | MT-7 | MT-8 | MT-9 | MT-10 | MT-11 | MT-12 | MT-13 | MT-14 | MT-15 | MT-16 | MT-17 |
Light hydrocarbon gas composition, in mole percent of dissolved gases | |||||||||||
71.01 | 0.0678 | 0.0100 | 0.0373 | 0.0079 | 0.0032 | <0.0001 | 0.0034 | 0.0128 | 0.0333 | 0.4650 | 12.74 |
0.0046 | <0.0001 | <0.0001 | <0.0001 | <0.0001 | 0.0004 | <0.0001 | <0.0001 | <0.0001 | <0.0001 | 0.0027 | 0.0046 |
<0.0001 | <0.0001 | <0.0001 | <0.0001 | <0.0001 | <0.0001 | <0.0001 | <0.0001 | <0.0001 | <0.0001 | <0.0001 | <0.0001 |
<0.0001 | <0.0001 | <0.0001 | <0.0001 | 0.0001 | <0.0001 | <0.0001 | <0.0001 | <0.0001 | <0.0001 | 0.0013 | 0.0034 |
<0.0001 | <0.0001 | <0.0001 | <0.0001 | <0.0001 | <0.0001 | <0.0001 | <0.0001 | <0.0001 | <0.0001 | 0.0004 | <0.0001 |
<0.0001 | <0.0001 | <0.0001 | <0.0001 | <0.0001 | <0.0001 | <0.0001 | <0.0001 | <0.0001 | <0.0001 | <0.0001 | <0.0001 |
<0.0001 | <0.0001 | <0.0001 | <0.0001 | <0.0001 | <0.0001 | <0.0001 | <0.0001 | <0.0001 | <0.0001 | 0.0004 | 0.0006 |
<0.0001 | <0.0001 | <0.0001 | <0.0001 | <0.0001 | <0.0001 | <0.0001 | <0.0001 | <0.0001 | 0.0005 | <0.0001 | <0.0001 |
<0.0001 | <0.0001 | <0.0001 | <0.0001 | <0.0001 | <0.0001 | <0.0001 | <0.0001 | <0.0001 | <0.0001 | 0.0009 | 0.0006 |
<0.0001 | <0.0001 | <0.0001 | <0.0001 | <0.0001 | <0.0001 | <0.0001 | <0.0001 | <0.0001 | <0.0001 | <0.0001 | <0.0001 |
Light hydrocarbon gas concentration, in milligrams per liter | |||||||||||
23 | 0.0140 | 0.0018 | 0.0069 | 0.0085 | 0.0009 | <0.0002 | 0.0009 | 0.0041 | 0.0062 | 0.1000 | 2.5 |
0.0029 | <0.0002 | <0.0002 | <0.0002 | <0.0002 | 0.0002 | <0.0002 | <0.0002 | <0.0002 | <0.0002 | 0.0012 | 0.0018 |
<0.0002 | <0.0002 | <0.0003 | <0.0002 | 0.0003 | <0.0002 | <0.0002 | <0.0002 | <0.0002 | <0.0002 | 0.0008 | 0.0019 |
Other light hydrocarbon gas properties | |||||||||||
15,437 | nc | nc | nc | 79 | 8 | nc | nc | nc | nc | 116 | 1,593 |
−77.70 | na | na | na | na | na | na | na | na | na | na | −66.73 |
−240.3 | na | na | na | na | na | na | na | na | na | na | −333.8 |
Table 3.7.
Summary of selected inorganic constituent concentrations for Regional Monitoring Program groundwater samples in the Montebello Oil Field study area, 2014 and 2017–18, historical groundwater samples, and historical oil-field water samples (California State Water Resources Control Board, 2017a, 2017b; California Department of Water Resources, 2018; California State Water Resources Control Board Division of Drinking Water, 2018; Water Replenishment District of Southern California, variously dated, 2019b; Metzger and others, 2020; U.S. Geological Survey, 2021).[RMP, Regional Monitoring Program; TDS, total dissolved solids; mg/L, milligram per liter; ND, not detected above laboratory detection level; —, no data; µg/L, microgram per liter]
Table 3.8.
Constituents with a concentration greater than its water-quality benchmark in the Montebello Oil Field study area, 2014 and 2017–18.[SMCL-US, U.S. Environmental Protection Agency (EPA) secondary maximum contaminant level; SMCL-CA, California secondary maximum contaminant level; HAL-US, EPA lifetime health advisory; HBSL-NC, U.S. Geological Survey noncancer health-based screening level; MCL-US, EPA maximum contaminant level; MCL-CA, California maximum contaminant level; —, none]
Sample identifier (fig. 1) |
Constituents with a concentration greater than a non-enforceable water-quality benchmark
(SMCL-US1, SMCL-CA2, HAL-US1, or HBSL-NC3) |
Constituents with a concentration greater than an enforceable water-quality benchmark
(MCL-US1 or MCL-CA2) |
---|---|---|
MTE-1 | Specific conductance, chloride, total dissolved solids | — |
MTE-2 | Manganese | — |
BNE-2 | Manganese | — |
BNE-3 | Specific conductance, ammonium, chloride, manganese, strontium, total dissolved solids, iron | Turbidity |
MT-1 | Cobalt | — |
MT-2 | — | — |
MT-3 | — | — |
MT-4 | — | — |
MT-5 | Cobalt | — |
MT-6 | — | — |
MT-7 | — | — |
MT-8 | Cobalt, manganese, iron | Turbidity |
MT-9 | pH | Arsenic |
MT-10 | Manganese | — |
MT-11 | — | — |
MT-12 | — | Trichloroethene (TCE) |
MT-13 | Manganese | — |
MT-14 | Manganese, iron | — |
MT-15 | Nickel | Turbidity |
MT-16 | Manganese, iron | Tetrachloroethene (PCE), trichloroethene (TCE), cis-1,2-dichloroethene |
MT-17 | Manganese, iron | Turbidity |
References Cited
California Department of Conservation, 2019b, Well search: California Department of Conservation web page, accessed December 2019 at https://secure.conservation.ca.gov/WellSearch.
California Department of Conservation, 2020c, Oil and gas online data: California Department of Conservation, Geologic Energy Management Division web page, accessed January 8, 2020, at https://www.conservation.ca.gov/calgem/Online_Data/Pages/Index.aspx.
California Department of Water Resources, 2018, Water data library (WDL) station map: California Department of Water Resources web page, accessed August 2018 at https://www.water.ca.gov/waterdatalibrary/waterquality/index.cfm/.
California State Water Resources Control Board, 2017a, GeoTracker electronic deliverable format (EDF) data: California State Water Resources Control Board web page, accessed May 2017 at https://geotracker.waterboards.ca.gov/data_download_by_county.
California State Water Resources Control Board, 2017b, GeoTracker GAMA web page: California State Water Resources Control Board, accessed July 17, 2017, at https://geotracker.waterboards.ca.gov/gama/.
California State Water Resources Control Board, 2019c, California regulations related to drinking water: California State Water Resources Control Board web page, accessed March 26, 2020, at https://www.waterboards.ca.gov/drinking_water/certlic/drinkingwater/Lawbook.shtml.
California State Water Resources Control Board Division of Drinking Water, 2018, EDT library and water quality analyses data and download page: California State Water Resources Control Board Division of Drinking Water digital data, accessed June 19, 2018, at https://www.waterboards.ca.gov/drinking_water/certlic/drinkingwater/EDTlibrary.shtml.
California State Water Resources Control Board Division of Drinking Water, 2020, Drinking water notification levels and response levels—An overview: California State Water Resources Control Board Division of Drinking Water, 16 p., accessed December 7, 2020, at https://www.waterboards.ca.gov/drinking_water/certlic/drinkingwater/NotificationLevels.html.
DeSimone, L.A., McMahon, P.B., and Rosen, M.R., 2014, The quality of our Nation’s waters—Water quality in Principal Aquifers of the United States, 1991–2010: U.S. Geological Survey Circular 1360, 151 p. [Available at https://doi.org/10.3133/cir1360.]
Metzger, L.F., Morris, D., and Watson, E., 2020, Historical produced water chemistry data compiled for selected oil fields in Los Angeles and Orange Counties, southern California: U.S. Geological Survey data release, accessed March 30, 2020, at https://doi.org/10.5066/P9JXQ3SD.
U.S. Environmental Protection Agency, 2019, Aquifer exemptions in the underground injection control program: U.S. Environmental Protection Agency web page, accessed December 2019 at https://www.epa.gov/uic/aquifer-exemptions-underground-injection-control-program.
U.S. Geological Survey, 2018, Health-based screening levels for evaluating water-quality data: U.S. Geological Survey web page, accessed on December 7, 2020, at https://water.usgs.gov/water-resources/hbsl/.
U.S. Geological Survey, 2021, USGS water data for the Nation: U.S. Geological Survey National Water Information System database, accessed January 12, 2021, at https://doi.org/10.5066/F7P55KJN.
Water Replenishment District of Southern California, variously dated, Regional groundwater monitoring report: Lakewood, Calif., Water Replenishment District of Southern California web page, accessed May 16, 2019, at https://www.wrd.org/regional-groundwater-monitoring-report.
Water Replenishment District of Southern California, 2019b, Well search tool: Water Replenishment District of Southern California web page, accessed May 2019 at https://gis.wrd.org/.
Appendix 4. Oil and Gas Well Annular Cement Calculations
Reference Cited
PetroWiki, 2015, Cement composition and classification: PetroWiki web page, accessed July 16, 2021, at https://petrowiki.spe.org/Cement_composition_and_classification.
Conversion Factors
International System of Units to U.S. customary units
Temperature in degrees Celsius (°C) may be converted to degrees Fahrenheit (°F) as follows:
°F = (1.8 × °C) + 32.
Datum
Vertical coordinate information is referenced to the North American Vertical Datum of 1988 (NAVD 88).
Horizontal coordinate information is referenced to the North American Datum of 1983 (NAD 83).
Altitude, as used in this report, refers to distance above the vertical datum.
Supplemental Information
Specific conductance is given in microsiemens per centimeter at 25 degrees Celsius (µS/cm at 25 °C).
Concentrations of chemical constituents in water are given in either milligrams per liter (mg/L) or micrograms per liter (µg/L).
Results for measurements of stable isotopes of an element (with symbol E) in water, solids, and dissolved constituents commonly are expressed as the relative difference in the ratio of the number of the less abundant isotope (iE) to the number of the more abundant isotope of a sample with respect to a measurement standard.
Tritium is given in tritium units (TU). A TU is equal to 3.19 picocuries per liter.
Carbon-14 in dissolved inorganic carbon is given in percent modern carbon (pmc).
The element helium (He) has atoms with different masses: Helium-3 (3He) has two protons and one neutron.
Dissolved light hydrocarbon gases are given in milligrams per liter (mg/L) or percentage of total dissolved gases (mole percent).
Abbreviations
14C-DIC
carbon-14 in dissolved inorganic carbon
δ2H-CH4
stable isotopes of hydrogen in methane
δ13C-CH4
stable isotopes of carbon in methane
3H
tritium
87Sr/86Sr
strontium-87/strontium-86
SF6
sulfur hexafluoride
API
American Petroleum Institute
bls
below land surface
BTEX
benzene, toluene, ethylbenzene, and xylenes
CalGEM
California Department of Conservation, Division of Geologic Energy Management
DIC
dissolved inorganic carbon
EOR
enhanced oil recovery
EPA
U.S. Environmental Protection Agency
LDL
laboratory detection level
OII
Operating Industries, Inc.
PCE
tetrachloroethylene
RMP
Regional Monitoring Program
TCE
trichloroethylene
USGS
U.S. Geological Survey
VOC
volatile organic compound
For more information concerning the research in this report, contact the
Director, California Water Science Center
U.S. Geological Survey
6000 J Street, Placer Hall
Sacramento, California 95819
https://www.usgs.gov/centers/ca-water/
Publishing support provided by the U.S. Geological Survey
Science Publishing Network, Sacramento Publishing Service Center
Suggested Citation
Stanton, J.S., Land, M., Landon, M.K., Shimabukuro, D.H., McMahon, P.B., Davis, T.A., Hunt, A.G., and Sowers, T.A., 2023, Groundwater quality near the Montebello Oil Field, Los Angeles County, California: U.S. Geological Survey Scientific Investigations Report 2022–5128, 80 p., https://doi.org/10.3133/sir20225128.
ISSN: 2328-0328 (online)
Study Area
Publication type | Report |
---|---|
Publication Subtype | USGS Numbered Series |
Title | Groundwater quality near the Montebello Oil Field, Los Angeles County, California |
Series title | Scientific Investigations Report |
Series number | 2022-5128 |
DOI | 10.3133/sir20225128 |
Year Published | 2023 |
Language | English |
Publisher | U.S. Geological Survey |
Publisher location | Reston, VA |
Contributing office(s) | California Water Science Center, Crustal Geophysics and Geochemistry Science Center |
Description | Report: ix, 80 p.; Data Release |
Country | United States |
State | California |
Other Geospatial | Montebello Oil Field |
Online Only (Y/N) | Y |
Google Analytic Metrics | Metrics page |