Response in the Water Quality of Delavan Lake, Wisconsin, to Changes in Phosphorus Loading—Setting New Goals for Loading from its Drainage Basin
Links
- Document: Report (2.4 MB pdf) , HTML , XML
- Dataset: USGS National Water Information System database —USGS water data for the Nation
- Data Release: USGS data release - Eutrophication models to simulate changes in the water quality of Green Lake, Wisconsin in response to changes in phosphorus loading, with supporting water-quality data for the lake, its tributaries, and atmospheric deposition
- Download citation as: RIS | Dublin Core
Acknowledgments
The authors would like to thank members of the Town of Delavan–Lake Committee, especially cochairs Mary Knipper and Katherine Gaulke, for supporting the overall project and Delavan Lake Sanitary District staff, especially administrator Jim DeLuca for supporting the tributary studies component of the study, and Charlie Handel for assistance with tributary and lake sampling.
We would like to thank Jerry Goddard of the U.S. Geological Survey for collecting much of the early tributary and lake data. We also would like to thank Lisa Lucas of the U.S. Geological Survey and Dale Buser of the Southeastern Wisconsin Regional Planning Commission for reviewing an earlier draft of this report and providing helpful comments.
Abstract
During 1989–92, an extensive rehabilitation project was completed in and around Delavan Lake, Wisconsin, to improve the lake’s water quality. However, in 2016, the lake was listed by the Wisconsin Department of Natural Resources as impaired for excessive algal growth (high chlorophyll a concentrations), and high phosphorus input was listed as its likely cause. In addition, the recent (2017–21) mean summer water clarity (as measured with a Secchi disk) was shallower than the goal set by the community (3.0 meters). Based primarily on flow and water-quality data collected in Jackson Creek, which is the main tributary of the lake, the mean annual phosphorus loading to the lake during water years (WYs) 2017–21 was 6,570 kilograms per year (kg/yr), and 306 kg/yr came from uncontrollable sources (atmospheric deposition and groundwater). Phosphorus loading during these years was about 48 percent higher than the long-term mean loading from WY 1984 to WY 2021. Based on results from Canfield-Bachmann phosphorus models, Carlson trophic state index relations, and the Jones and Bachmann chlorophyll a relation, external phosphorus loading would need to be decreased from 6,570 to 5,270 kg/yr (a 21-percent reduction in the potentially controllable external phosphorus load from the base period of WYs 2017–21) for chlorophyll a concentrations greater than 20 micrograms per liter to be detected no more than 5.0 percent of the time (the Wisconsin Department of Natural Resources criterion for chlorophyll a impairment for the lake). Based on Carlson trophic state index relations, external loading would need to be decreased from 6,570 to 4,380 kg/yr (a 35-percent reduction in the potentially controllable external phosphorus load) for summer mean Secchi depths to increase to 3.0 meters. Therefore, for Delavan Lake to reach the water-quality criteria for impairment and the goals for all three water-quality constituents, a 35-percent reduction in the potentially controllable phosphorus load is needed, which equates to a reduction in total phosphorus loading from 6,570 to 4,380 kg/yr. A 35-percent reduction in phosphorus loading to improve the water quality of Delavan Lake is less than the 49-percent reduction in phosphorus loading required for the area near Delavan Lake to improve the water quality of the Rock River and its tributaries indicated in the Rock River total maximum daily load.
Introduction
In the mid-1800s, early settlers described Delavan Lake (fig. 1) as a beautiful, pristine lake with excellent water quality and many game fish (University of Wisconsin-Madison and others, 1986). As with many freshwater lakes in agricultural and urban areas, the water quality in Delavan Lake declined because of cultural eutrophication from excessive phosphorus input from point and nonpoint sources in its drainage basin (Schindler and others, 2016). Before 1980, Delavan Lake was ranked one of the worst lakes in the State of Wisconsin in terms of water quality, and the lake experienced frequent algal blooms and a fishery dominated by rough fish (University of Wisconsin-Madison and others, 1986). To improve the lake’s water quality, one of the most comprehensive lake rehabilitation plans ever developed was implemented to shift the lake from a hypereutrophic condition back to a mesotrophic condition and improve the lake’s fishery (Robertson and others, 2000). The plan was to treat the sources of the problems (reduce external and internal phosphorus loading and rehabilitate the fishery) rather than treat the individual symptoms observed. Each of the management actions (point-source reduction, food-web manipulations, alum treatment, and nutrient load reduction strategies including detention pond construction on the main tributary to the lake) is described in detail by Robertson and others (2000). In addition, since the completion of the rehabilitation project, the detention ponds upstream from Mound Road and the inlet (fig. 1) have been dredged to remove sediment and phosphorus during 2007 and 2011, respectively. Each physical, chemical, and biological component of the plan had varying degrees of success over different periods. The timing of each management action and the response in near-surface total phosphorus (TP) concentrations are shown in figure 2. As a result of meteorological variability (floods and extended droughts) and implementation of the various management strategies, near-surface TP concentrations in the lake have fluctuated, but mean TP concentrations gradually decreased to below the goal established for the lake in the rehabilitation plan (0.034 milligram per liter [mg/L]; University of Wisconsin-Madison and others, 1986). More than 30 years since the original rehabilitative efforts began, TP concentrations, water clarity, algal concentrations, and the fishery in the lake have improved from what they were like in the early 1980s.
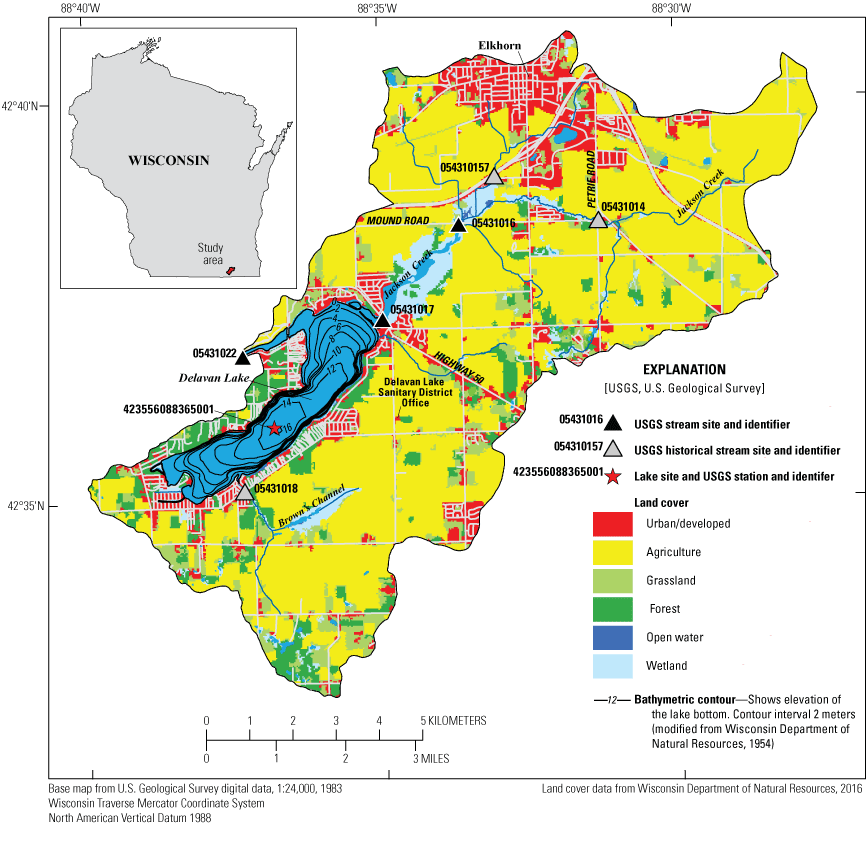
Delavan Lake, Wisconsin, and its drainage basin, with sampling locations and land use identified. Land-use classifications were defined from Wiscland 2.0 data (Wisconsin Department of Natural Resources, 2016). Lake bathymetric contours are in 2-meter increments. U.S. Geological Survey station identifiers are shown on the figure.
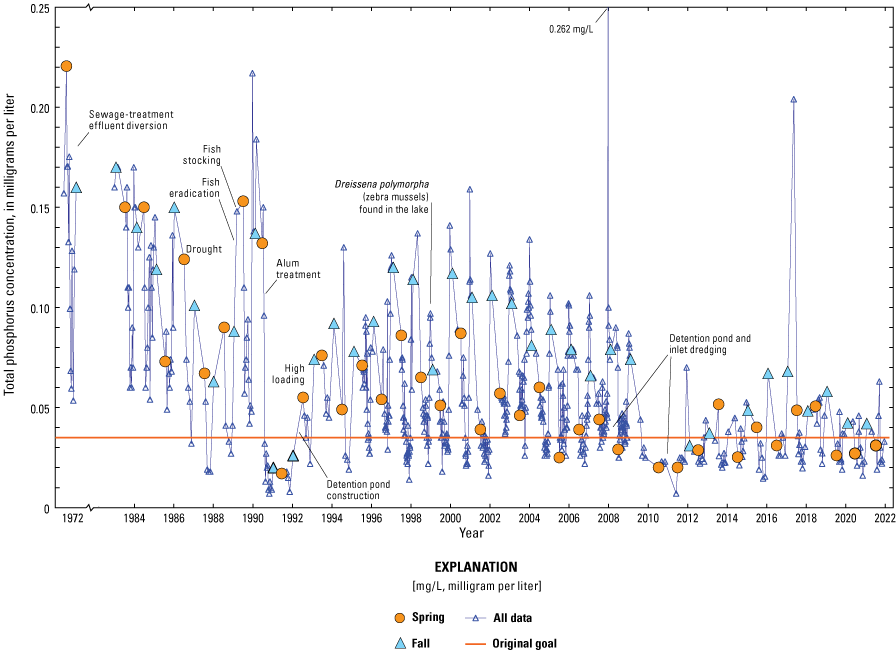
Near-surface total phosphorus concentrations in Delavan Lake, Wisconsin, from 1972 to 2022. Timings of specific management actions taken in Delavan Lake and its drainage basin, which are described in the text, are identified on the graph.
In 2016, however, Delavan Lake was listed as impaired for excessive algal growth, and high phosphorus input was listed as its likely cause (Wisconsin Department of Natural Resources, 2021). The TP and algal (chlorophyll a [Chl a]) concentration criteria for impairment established by the Wisconsin Department of Natural Resources (WDNR) for stratified drainage lakes, like Delavan Lake, are a 5-year geometric mean TP concentration between June 1 and September 15 of 0.030 mg/L and Chl a concentrations less than 20 micrograms per liter (μg/L) occurring on 95 percent of the days between July 15 and September 15 (Wisconsin Department of Natural Resources, 2019). A geometric mean is a measure of central tendency that is often used with nonnormally distributed data and is computed by taking the mean of the logarithm of the original values and then converting the mean logarithmic value back to the original units. The criterion for TP impairment is less than the original 0.034-mg/L TP-concentration goal for the lake. In 2021, the Town of Delavan–Lake Committee also formally set a water-clarity goal for the lake of a mean Secchi depth (SD) of at least 3 meters (m) during July through September, with a secondary goal of a mean SD during July and August to be at least 3 m (M. Knipper, Town of Delavan–Lake Committee, written commun., 2021). The original water-clarity goal for Delavan Lake was a summer mean of 1.5 m (University of Wisconsin-Madison and others, 1986). Reaching these criteria for impairment and goals requires additional management strategies (phosphorus-load reductions) to be developed and implemented for the lake and its drainage basin. Several plans have been published during the past few years that provide guidance on specific actions that can be taken to reduce phosphorus loads to Delavan Lake (Berrini & Associates, LLC, and Northwater Consulting, 2016; Southeastern Wisconsin Regional Planning Commission, 2017). Additionally, the Delavan Lake Comprehensive Management Plan (Southeastern Wisconsin Regional Planning Commission, 2002) is currently being updated, and plans include a prioritized list of specific actions to be taken to reduce phosphorus loads to Delavan Lake.
Delavan Lake is in the Rock River drainage basin. Many streams and rivers in the Rock River drainage basin are considered impaired for phosphorus and sediment. Therefore, a total maximum daily load (TMDL) was prepared for the Rock River drainage basin by the Cadmus Group (2011). As part of the Rock River TMDL, it was indicated that the area near Delavan Lake should reduce its nonpoint phosphorus load by 49 percent from the mean annual loading during 1989–98 to delist the streams and rivers downstream from the lake.
In this report, we describe the changes in the water quality (TP, Chl a, and SD) of Delavan Lake from 1972 to 2022 and the changes in the phosphorus loading to the lake during water years (WYs) 1984–2021. Various water-quality models are then used to develop response curves for TP, Chl a, and SD that can be used to develop management plans for the lake that, if followed, could improve the water quality in the lake and remove it from being considered impaired by the WDNR.
Study Site
Delavan Lake is a dimictic (stratifies during summer and winter), eutrophic lake in southeastern Wisconsin. The lake has a surface area of 725 hectares, a mean depth of 7.6 m, and a maximum depth of 16.5 m (Field and Duerk, 1988). The lake has a 99.6-square-kilometer drainage basin that is predominantly agricultural (60.8 percent), but it does include the city of Elkhorn (based on land-use/land-cover data from the Wisconsin Department of Natural Resources [2016]). Soils in the Delavan Lake drainage basin are mostly clay, silt, sandy clay loams, and silty clay loams (Haszel, 1971), which are primarily classified as moderately well drained (71 percent) and poorly drained organic and clay soils (17 percent; Southeastern Wisconsin Regional Planning Commission, 2002). In 1969, the Delavan Lake Sanitary District (DLSD) was formed, and by 1981, a complete sanitary sewer system was installed to remove septic-tank inputs and divert effluent from the Delavan Lake drainage basin. Therefore, since 1981, most of the external phosphorus loading to the lake is believed to come from nonpoint-source tributary loading. There are two main tributaries to Delavan Lake: Jackson Creek and Brown’s Channel (fig. 1). The main tributary, Jackson Creek, which represents about 56 percent of the drainage basin, contributed about 69 percent of the basin’s water load and about 79 percent of the basin’s phosphorus load based on detailed hydrologic and phosphorus budgets constructed during WYs 1984–85 by Field and Duerk (1988).
Recognizing the substantial contribution from Jackson Creek to the overall phosphorus load delivered to Delavan Lake, a detailed watershed management plan prepared during 2017 focused on Jackson Creek (Southeastern Wisconsin Regional Planning Commission, 2017). This plan provided specific recommendations to reduce the phosphorus load from Jackson Creek by 44 percent.
General Approach
The general approach used in this study is as follows.
-
1. Describe the historical water quality (near-surface TP and Chl a concentrations and SD) in the lake (1972–2022) and use those data to describe the baseline conditions (mean summer) for the lake (5 years from 2017 to 2021). Baseline conditions reflect the current water-quality conditions in the lake.
-
2. Describe the new water-quality goals for Delavan Lake. These goals are to reflect the water quality required to remove the lake from the WDNR Impaired Waters List (Wisconsin Department of Natural Resources, 2021) and meet the goals established by the Town of Delavan-Lake Committee.
-
3. Develop response curves for near-surface mean summer TP and Chl a concentrations and SD based on data recently collected for the lake (for the 2017–21 base period). The response curves reflect how each of these constituents should respond to changes in phosphorus loading to the lake given the biological conditions (such as food-web structure: plankton, Dreissena polymorpha [zebra mussels], and fishery in the lake).
-
4. Use the response curves to determine the total and potentially controllable phosphorus loading to the lake needed to reach the TP and Chl a concentrations and SD goals for the lake.
Methods of Data Collection, Flow and Load Estimation, and Eutrophication Modeling
This section of the report describes the methods used for data collection and compilation of lake water-quality (TP, Chl a, and SD), streamflow, stream water-quality (TP), stream phosphorus loads, meteorologic input (precipitation), and atmospheric phosphorus input data. Methods used to determine water and phosphorus inputs from unmonitored areas around the lake are described in this section. Statistical eutrophication models used to describe the response of the lake (TP, Chl a, and SD) to changes in phosphorus loading also are described.
Lake Water-Quality Monitoring
Routine monitoring of Delavan Lake and its drainage basin by the U.S. Geological Survey (USGS) began as part of a diagnostic study in WY 1984 (Field and Duerk, 1988) and has continued to the present (2023). This monitoring provides data to describe the water quality in the lake and the computation of tributary phosphorus loading to the lake. Annual monitoring of the lake typically consisted of monthly sampling from April through September, one-time sampling during fall turnover (October or November), and one-time sampling during midwinter (February). Primary sampling was done at the center of the lake near its deepest location (USGS station 423556088365001), although additional secondary sampling has been done on the north and south ends of the lake during some years. During each sampling, water temperature, dissolved oxygen, specific conductance, and pH profiles were collected with a multiparameter meter, and SDs were measured with a 20-centimeter (cm) black and white Secchi disk. Water samples were routinely collected near the surface (0.5 m) for TP, dissolved orthophosphate, and Chl a concentrations, in addition to other water-quality constituents. Water samples were collected with a Van Dorn sampler. Additional TP concentrations were measured in late summer to estimate the phosphorus contributed to the lake from internal recycling. For further details on USGS sampling techniques, see Manteufel and others (2012). Only the water-quality data collected near the surface of the lake were used in this study. All chemical analyses were completed by the Wisconsin State Laboratory of Hygiene in accordance with standard analytical procedures (Wisconsin State Laboratory of Hygiene, 2020). All lake and stream water-quality data, streamflow data, and phosphorus loads computed for various stream locations are available from the USGS National Water Information System database (U.S. Geological Survey, 2022) and can be accessed by using the applicable USGS station numbers provided in this report.
In addition to monitoring by the USGS, TP, Chl a, and SD have been measured near the deepest location in the lake by staff of the DLSD as part of the WDNR Citizen Lake Monitoring Program (Wisconsin Department of Natural Resources, 2022a). DLSD sampling typically consisted of monthly sampling for TP and Chl a during June through August and twice per month sampling for SD during April through October. During each visit, consistent protocols were followed (University of Wisconsin-Stevens Point, 2022). All chemical analyses were completed by the Wisconsin State Laboratory of Hygiene. These data were obtained from the WDNR Surface Water Integrated Monitoring System database using the WDNR online retrieval tool (Wisconsin Department of Natural Resources, 2022b). In addition, TP and Chl a concentrations and SDs were measured near the deepest location in the lake during March–October 1972 (Stauffer, 1974; daily data are contained in this report). Stauffer used a 25-cm black and white Secchi disk. A 1.043 conversion was used to adjust the SD data to be equivalent to data measured with a 20-cm black and white disk (Lathrop, 1992).
One method of classifying the water quality of a lake is with trophic state index (TSI) values based on near-surface concentrations of TP and Chl a and SD, as developed by Carlson (1977). The indices were developed to place these three characteristics on similar scales to allow trophic comparisons among constituents. TSI values based on TP (TSITP), Chl a (TSIChl a), and SD (TSISD) were computed using equations 1–3, respectively. Summer mean TSI values were computed using the mean of the mean monthly concentrations or depths from the reference period for each constituent: TP (June–September), Chl a (July–September), and SD (July–September). Different periods were used for each of the constituents to be consistent with the WDNR evaluation periods.
whereLake Morphometry
Morphometric characteristics of Delavan Lake were reevaluated based on the 1954 contour map produced by the Wisconsin Conservation Department (Wisconsin Department of Natural Resources, 2020). The surface area of the lake was adjusted on the basis of an aerial image obtained from the 2005 National Agriculture Imagery Program (U.S. Department of Agriculture, 2006). The area and volume of the lake at specific depths were computed from the depth contours of the map using a geographic information system. The area, volume, and mean depth are 6,964,000 square meters, 52,960,000 cubic meters (m3), and 7.61 m, respectively; these values do not include the inlet to or outlet from the lake.
Stream Monitoring
Several tributary sites in the Jackson Creek basin (fig. 1) were instrumented to estimate the total daily flow into Delavan Lake from Jackson Creek at Highway 50 (USGS station 05431017). Because of financial constraints, the operation of these streamgages varied through time, which meant that the approach to estimate total flow from Jackson Creek also varied. During WYs 1983–92, daily flows at Highway 50 were estimated by extrapolating the flows measured at Jackson Creek at Petrie Road near Elkhorn (JCP, USGS station 05431014) and Jackson Creek Tributary near Elkhorn (USGS station 054310157; fig. 1). Flows at these sites were continuously estimated by monitoring stage and the use of stage-discharge relations. Streamflow at Highway 50 was estimated by summing the flows at these two sites and flow from the ungaged area (estimated by use of a drainage-area ratio between the ungaged area and the area upstream from JCP multiplied by the flow at JCP). Daily streamflows at Highway 50 during WYs 1993–95 were estimated by summing the flows measured at Jackson Creek at Mound Road near Elkhorn (USGS station 05431016), which was estimated by monitoring stage and using a stage-discharge relation, and flow from the ungaged area downstream from Mound Road (estimated using a drainage-area ratio between the ungaged area plus the area upstream from JCP multiplied by the flow measured at JCP). The flow at JCP was used to extrapolate flows from the ungaged area because the area upstream from JCP had similar environmental characteristics to the ungaged area. During this time, the median daily extrapolation factor for the area downstream of Mound Road was 1.3. Streamflows at Highway 50 during WYs 1993–2006 were estimated by multiplying flows measured at Jackson Creek at Mound Road by 1.3, which is the ratio in their drainage areas. Streamflows at Highway 50 during WYs 2007–9 were measured directly at Highway 50 using an acoustic Doppler velocity meter and water-level sensor (Sauer, 2002) because backwater conditions did not enable flow to be estimated solely from changes in water elevation. During this time, the median daily extrapolation factor for flows measured at Mound Road to Highway 50 was 1.4. No flows were measured during WYs 2010–11. Streamflows at Highway 50 during WYs 2012–21 were estimated by multiplying flows measured at Jackson Creek at Mound Road by 1.4.
Samples for water-quality analyses were manually collected from the bridge on Highway 50 using a DH–59 sampler (U.S. Geological Survey, 2018). The mean number of samples collected per year during WYs 2017–21 was 27 (ranging from 18 to 34), and the mean number of samples collected per year for WYs 1984–2021 was 87. Water samples also were collected at Mound Road during WYs 1993–2021 using automated samplers and augmented with routine, manually collected base-flow samples using a DH–59 sampler. The mean number of samples collected per year at Mound Road during WYs 2017–21 was 90. All chemical analyses were completed by the Wisconsin State Laboratory of Hygiene in accordance with standard analytical procedures (Wisconsin State Laboratory of Hygiene, 2020).
Daily phosphorus loads (summarized to annual loads) were computed at all sites using integration methods described by Porterfield (1972), including the graphical constituent loading analysis system (Koltun and others, 2006). The graphical constituent loading analysis system and the other integration methods were developed to estimate constituent loads from continuous measurements of streamflow and instantaneous constituent concentrations. To obtain continuous estimations of concentration, concentrations were linearly interpolated between measurements except at the beginning and end of events with large changes in flow. Before interpolations, additional estimated concentrations were often added to the time series to better describe the concentrations just before and after an event or to describe events without measured concentrations. The filled-in concentrations at the beginning of an event were typically estimated from concentrations measured during previous low-flow periods. Concentrations at the end of the events were typically estimated from concentrations measured in that stream shortly after the end of an event. The more frequent concentrations measured at Mound Road also were used to estimate additional concentrations at Highway 50, especially during unsampled events. All flow, stream water-quality, and load data can be accessed from the USGS National Water Information System database (U.S. Geological Survey, 2022) by using the USGS station numbers.
Unmonitored Water and Phosphorus Inputs
Inputs of water and phosphorus from the drainage areas around Delavan Lake, not including those from Jackson Creek, are referred to as “inputs from unmonitored areas,” which represent about 44 percent of the total drainage basin. Annual flows from the unmonitored areas were estimated from information from a detailed water budget constructed for the lake during WYs 1984–85 by Field and Duerk (1988). Based on the annual water budgets for WYs 1984–85, the average annual flows from the unmonitored areas were equal to the flow measured at Highway 50 times 0.443.
Inputs of phosphorus from the unmonitored areas in WYs 1984 and 1985 were obtained directly from Field and Duerk (1988). During WYs 1984–85, the mean annual TP concentration from this area was estimated to be 0.177 mg/L. Annual inputs of phosphorus from the unmonitored areas during WYs 1986–99 were obtained by multiplying the estimated annual flow from the unmonitored area by 0.177 mg/L. After WYs 1984–85, efforts were made to reduce the phosphorus loss from its drainage basin; however, the effects of these efforts were not quantified. An estimated 43-percent decrease in TP concentrations at Highway 50 was probably the result of earlier decreases in point-sources loads in the basin (Robertson and others, 2000) and efforts in the basin to reduce nonpoint phosphorus contributions. Therefore, it was assumed that after 1999, the mean TP concentration in the unmonitored areas, which did not have major point-source reductions, decreased by 25 percent (to 0.133 mg/L), which is about one-half the change in concentration measured at Highway 50 (see the “Water and Phosphorus Loading to Delavan Lake” section). Inputs of phosphorus from the unmonitored areas in WYs 2000–21 were obtained by multiplying the estimated annual flow from these areas by the 0.133 mg/L.
Precipitation
Daily precipitation on Delavan Lake was estimated from precipitation measured at a nearby weather station in Lake Geneva, Wis. (Midwest Regional Climate Center station 47445; https://mrcc.purdue.edu/CLIMATE/Station/Daily), from 1984 to 2003, which is about 7 kilometers southeast of Delavan Lake (Midwestern Regional Climate Center, 2022). This station was discontinued in 2003, so data from a different nearby station in Clinton, Wis. (Midwest Regional Climate Center station 471667; https://mrcc.purdue.edu/CLIMATE/Station/Daily), which is about 20 kilometers west of Delavan Lake, were used from 2003 to 2021.
Atmospheric Sources of Phosphorus
Atmospheric phosphorus inputs are not readily available for most areas of the United States. Therefore, atmospheric phosphorus inputs were measured as part of an earlier study near Delavan Lake. Atmospheric inputs of phosphorus were estimated from concentrations measured in wetfall (rain and snow) and dryfall (phosphorus deposition rates) during 2005–9 at a station installed near the DLSD office (fig. 1; Robertson and others, 2022). Deposition rates and TP concentrations were measured with a 30-cm-diameter stainless-steel collection bowl mounted on a table about 1 m above the ground. Netting was placed over the bowl to prevent contamination by birds. This equipment also was used to estimate atmospheric phosphorus inputs to Whitefish Lake in northwestern Wisconsin (Robertson and others, 2009). DSLD personnel serviced the monitoring station and collected wet samples and dry samples. For wet samples, the bowl was deployed within a few hours of an imminent precipitation event, and the samples were collected as soon as possible after the event to reduce evaporation and other contamination. Dry samples were those collected after several days, usually 7–10 days, without precipitation. Dry samples were taken from the bowl by rinsing the bowl twice with 125 milliliters of distilled water. Both types of samples were acidified and chilled before being sent to the Wisconsin State Laboratory of Hygiene for analysis of TP concentrations. The stainless-steel bowl was cleaned with phosphate-free soap before each deployment. Rates of phosphorus dryfall deposition were computed by determining the mass of phosphorus deposited (dry sample concentration×0.25 liter) per unit area per day of deployment. All wetfall concentrations were adjusted to remove the phosphorus from dryfall during its collection period by subtracting the mass of phosphorus deposited during the wetfall sample based on the monthly mean dryfall deposition rate and then dividing the resulting mass of phosphorus in the sample by its volume. Samples that included insects and other nondepositional material were discarded. All data collected for these analyses are available in Robertson and Kennedy (2021).
Numerical Eutrophication Models
A combination of empirical eutrophication models was used to estimate the response in the water quality in Delavan Lake to changes in phosphorus loading from its drainage basin. The Canfield-Bachmann natural-lake and artificial-lake models (Canfield and Bachmann, 1981) were first used to estimate how near-surface TP concentrations in Delavan Lake are expected to respond to changes in phosphorus loading using the estimated mean annual phosphorus loading during the base period of WYs 2017–21 and a series of 17 additional incremental changes in phosphorus loading (referred to as “scenarios”). Then, the simulated changes in TP concentrations (values from the TP response curve) were used to either (1) estimate the expected responses of Chl a concentrations and SDs using the relations in TSI values measured in the data from the lake during 2017–21 or (2) estimate the expected responses of Chl a concentrations using the Jones and Bachmann (1976) TP–Chl a relation. Finally, output from each model was calibrated (adjusted) to the conditions in Delavan Lake; in other words, the model output was adjusted for any biases during the base period. For these empirical models, there are no true calibration parameters; therefore, to account for model biases, all model results were adjusted by the percentage of bias in the results from the mean of the measured data from the base period (2017–21). This adjustment for model bias is consistent with that used in other studies (Robertson and others, 2009, 2018, 2022).
Canfield-Bachmann Total Phosphorus Models
Several empirical models that relate phosphorus loading to a lake to specific water-quality characteristics could be used to estimate how changes in phosphorus loading to Delavan Lake could affect its water quality. Most of these models were developed based on data from many different lake systems with specific measures describing lake water quality, such as near-surface mean summer TP and Chl a concentrations and SD and coinciding phosphorus-loading information. Two of these models are the Canfield-Bachmann natural-lake model (eq. 4) and artificial-lake model (eq. 5; Canfield and Bachmann, 1981), which both relate external phosphorus loading per unit area of the lake surface, hydrology (residence time), and mean lake depth to the near-surface mean summer TP concentration.
whereTP
is the near-surface mean summer total phosphorus concentration, in milligrams per liter;
LA
is the mean annual phosphorus-loading rate per unit area of the lake, in milligrams per square meter per year;
z
is the mean depth of the lake, in meters; and
τ
is the water residence time, in years.
Internal recycling of phosphorus from the sediments of the lake is not typically included as a source in most of these empirical models, including the Canfield-Bachmann models, because most models were developed without explicitly including this source (Canfield and Bachmann, 1981). Therefore, these models indirectly incorporate contributions from internal sediment recycling (Walker, 1996). Internal sediment recycling is typically only added as a source when a lake has abnormally high internal sediment recycling (Walker, 1996). Therefore, the Canfield-Bachmann models were applied to Delavan Lake without considering contributions from internal sediment recycling. This assumption should be valid because an alum layer buried in the sediments as part of the lake rehabilitation program (fig. 2) should reduce historically high phosphorus contributions (Robertson and others, 2000) and any new deposition most likely has a lower phosphorus concentration than the previous deposition.
To develop the response curves in TP concentrations to changes in phosphorus loading, the natural-lake and artificial-lake Canfield-Bachmann models were used to simulate near-surface mean summer TP concentrations in Delavan Lake using the mean annual phosphorus loading for WYs 2017–21 and 17 additional incremental annual phosphorus-load changes from −90 percent to +100 percent. In these 18 simulations, constant mean depth and residence time were assumed. To develop the final TP response curve, the results from these two models were averaged and then adjusted for any bias in model simulations for the base period of WYs 2017–21. Both the Canfield-Bachmann natural-lake and artificial-lake models were used because of the potential effects of the outlet dam.
Empirical Chlorophyll a and Secchi Disk Depth Models
During 2017–21, the summer mean TSI values for TP, Chl a, and SD in Delavan Lake had a relatively consistent relation. The TP response curve and the relations among mean TSI values and the TSI equations (eqs. 1–3) were used to simulate the response in Chl a and SD to the 18 incremental changes in mean annual phosphorus loading. To estimate the Chl a and SD response curves, simulated TP concentrations (bias-adjusted response curve predictions) from the Canfield-Bachmann models (eqs. 4 and 5) were first converted to TSITP values using equation 1. The TSITP values were then adjusted to TSIChl a and TSISD values using the relations determined between TSI values during 2017–21. The TSIChl a and TSISD values for each phosphorus-loading scenario were then converted back to Chl a concentrations in micrograms per liter and SDs in meters using equations 2 and 3. Finally, the simulated Chl a concentrations and SDs were adjusted by the percentage of bias in the results from the geometric mean of the measured data from the base period (2017–21).
The simulated TP concentrations (bias adjusted) from the Canfield-Bachmann models (eqs. 4 and 5) also were used to estimate the response in Chl a concentrations to changes in phosphorus loading using the Jones and Bachmann (1976) TP–Chl a relation (eq. 6) that is only a function of near-surface TP concentration. The simulated Chl a concentrations were then adjusted by the percentage of bias in the results from the geometric mean of the measured data from the base period (WYs 2017–21).
whereLake Water Quality
Near-surface TP concentrations have not only had large intra-annual variability but also have substantially changed in Delavan Lake from 1972 to 2022 following various management actions (Robertson and others, 2000) and multiyear stretches of atypical weather and related changes in phosphorus loads (fig. 2). Concentrations were generally highest during spring and fall turnover because of sediment phosphorus release. Before the diversion of sewage-treatment effluent from the Delavan Lake drainage basin that began in 1979 (Robertson and others, 2000), TP concentrations were often measured at greater than 0.15 mg/L. After the diversion, TP concentrations gradually declined until about 1988 (coinciding with a major drought in the area). Concentrations then increased quickly after the eradication of fish in the lake as part of the rehabilitation project (but without removal of the dead fish; Robertson and others, 2000). Concentrations then decreased to less than 0.02 mg/L in response to a lake-wide alum treatment (Robertson and others, 2000). Concentrations then gradually increased and fluctuated between about 0.025 and 0.13 mg/L from 1994 to about 2009. After dredging of the upstream constructed detention ponds in 2007–8 and the inlet region on Jackson Creek in 2011 (Berrini & Associates, LLC, and Northwater Consulting, 2016), TP concentrations declined to about 0.020 to 0.060 mg/L and generally fluctuated relatively little during 2012–22. Zebra mussels were first reported in the lake in 1999 (H. Bunk, Wisconsin Department of Natural Resources, written commun., 2014) but had little effect on TP concentrations. A few high TP concentrations were measured during algal blooms in the lake.
Annual summer mean TP concentrations (computed from the mean monthly concentrations for each month from June to September; fig. 3A; table 1) had a similar trend in concentrations to that observed in all the daily data shown in figure 2. Summer mean concentrations typically remained greater than the original 0.034-mg/L goal for the lake until 2010. Since 2010, summer mean concentrations have fluctuated around 0.030 mg/L, which is the criterion for TP impairment for stratified drainage lakes in Wisconsin (Wisconsin Department of Natural Resources, 2019). The geometric mean concentration computed from the individual data from June 1 to September 15 for the 2017–21 base period was 0.030 mg/L (table 1), which is similar to the WDNR criterion for impairment.
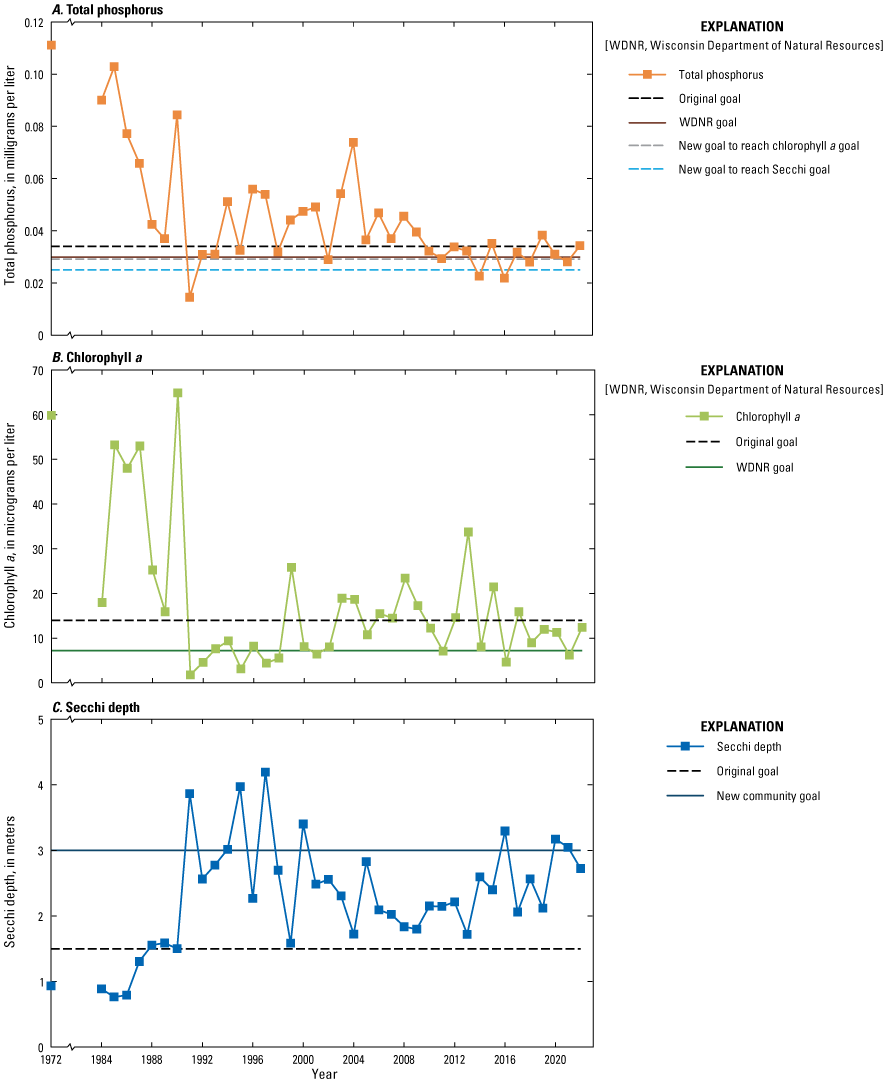
Annual summer mean near-surface (A) total phosphorus and (B) chlorophyll a concentrations and (C) Secchi depths in Delavan Lake, Wisconsin, 1972–2022. Specific goals and the Wisconsin Department of Natural Resources (WDNR) criterion for impairment (goal) for each constituent are identified.
Table 1.
Annual summer mean near-surface water quality in Delavan Lake, Wisconsin. Total phosphorus concentrations are for June–September, chlorophyll a concentrations are for July–September, and Secchi depths are for July–September. Trophic state index values were computed from summer mean water quality using equations 1–3 in the text.[TSI, trophic state index; NC, not computed]
Dissolved orthophosphate concentrations remained low in the epilimnion throughout summer, rarely exceeding 0.010 mg/L. Because dissolved concentrations remained very low throughout summer, phosphorus should be the nutrient that typically limits or controls the growth of algae. Therefore, controlling the phosphorus input to the lake also should control excessive algal growth in the lake.
Annual summer mean near-surface Chl a concentrations (computed from the mean monthly concentrations for each month from July to September—the period evaluated by the WDNR for Chl a concentrations) were typically greater than 20 µg/L before the rehabilitation project in 1989–92 (fig. 3B; table 1). Concentrations then dropped substantially during 1991–99, which was believed to be the result of the alum treatment and food-web manipulation (Robertson and others, 2000). Since 2000, summer mean Chl a concentrations ranged from about 5 to 34 µg/L. Zebra mussels were first reported in the lake in 1999 (H. Bunk, Wisconsin Department of Natural Resources, written commun., 2014). Based on the individual data collected within 1 week of July 15 to September 15 during the 2017–21 base period (Wisconsin Department of Natural Resources, 2019, p. 33), the geometric mean July–September Chl a concentration was 8.9 μg/L (table 1), and the standard deviation was 6.2 μg/L (or 0.62 natural logarithmic unit). Based on the mean and standard deviation, Chl a concentrations greater than 20 μg/L should be detected about 9.6 percent of the time (exceedance frequency), which is greater than the WDNR Chl a criterion for impairment of 5 percent of the time for stratified drainage lakes in Wisconsin. The exceedance frequency was computed as the percentage of the days in which the 20-μg/L specified value in logarithmic space was exceeded using the mean and standard deviation of the logarithms of the measured Chl a values (eq. 7). This value was computed using the NORMDIST function in Excel:
(7)
Based on the variability in Chl a concentrations (standard deviation of 0.62 natural logarithmic unit) observed in Delavan Lake during WYs 2017–21, the lake would be required to have a geometric mean Chl a concentration of 7.2 µg/L for concentrations greater than 20 µg/L to be detected no more than 5.0 percent of the time, which equates to a 19-percent reduction in all measured values.
The changes in annual summer mean water clarity in the lake as quantified using summer mean SDs (computed from the mean monthly data for each month from July to September values—the period evaluated by the WDNR for SD) were the inverse of that observed for TP and Chl a concentrations (fig. 3C). Annual summer mean SDs were typically less than 1.5 m before the rehabilitation project in 1989–92. Clarity then increased substantially in 1991, which again was believed to be caused by the alum treatment and food-web manipulation (Robertson and others, 2000). Since 1991, summer mean SDs ranged from about 1.5 to 4.2 m. The geometric mean July–September SD for the 2017–21 base period was 2.38 m, which is less than the 3.0-m goal established by the Delavan Lake community.
Changes in annual monthly mean SD values for each summer month (May through September; fig. 4) had a similar pattern to that of the summer mean SDs. In general, clarity was best in May and worst in July through September. The geometric mean July–August SD for the 2017–21 base period was 2.41 m, which is similar to that for July–September, 2.38 m, measured during the base period. Therefore, the phosphorus-load reduction needed to increase the mean summer water clarity to 3.0 m also should improve the mean July–August SD to greater than 3.0 m.
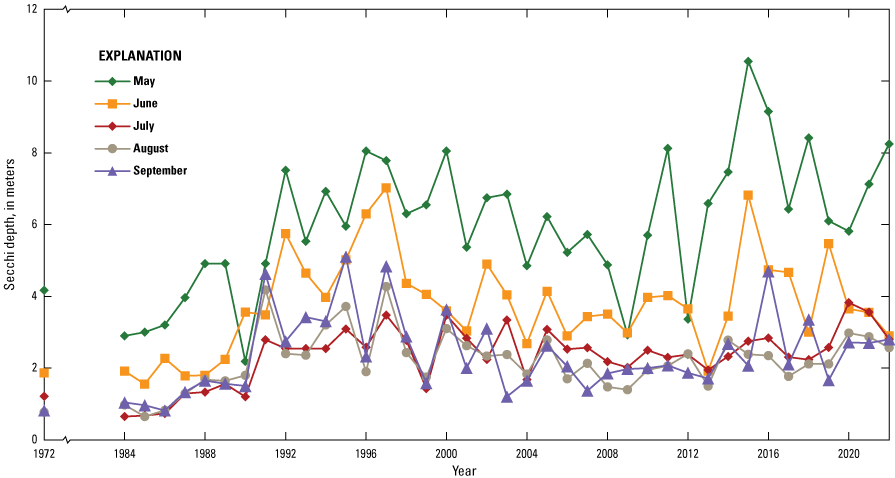
Annual monthly mean Secchi depths for May through September in Delavan Lake, Wisconsin, 1972–2022.
Based on the trophic state index values computed from the summer mean near-surface TP and Chl a concentrations, and SDs, Delavan Lake was classified as eutrophic (TSI values greater than 50) in the 1970s and 1980s based on all three constituents (fig. 5). Then, during and shortly after the rehabilitation project (1991), the lake was classified as oligotrophic or nearly oligotrophic (TSI values less than 40) based on all three constituents. Between 1992 and 2022, the trophic status of the lake depends on which constituent is being considered. Based on TP and Chl a, the lake was eutrophic, except in a few years when it was classified as mesotrophic (TSI values between 40 and 50). Based on SD, the lake was almost always mesotrophic or barely eutrophic. Since about 2005, the relation among the summer TSI values has been relatively consistent with TSISD greater than TSITP and TSIChl a. Part of the difference in TSI values may be related to zebra mussels being introduced in the lake around 1999 and other changes in the structure of the food web. During the 2017–21 base period, the mean TSIChl a was 0.003 times higher than the mean TSITP (TSIChl a=TSITP×1.003), and the mean TSISD was 0.859 times the mean TSITP (TSISD=TSITP×0.859).
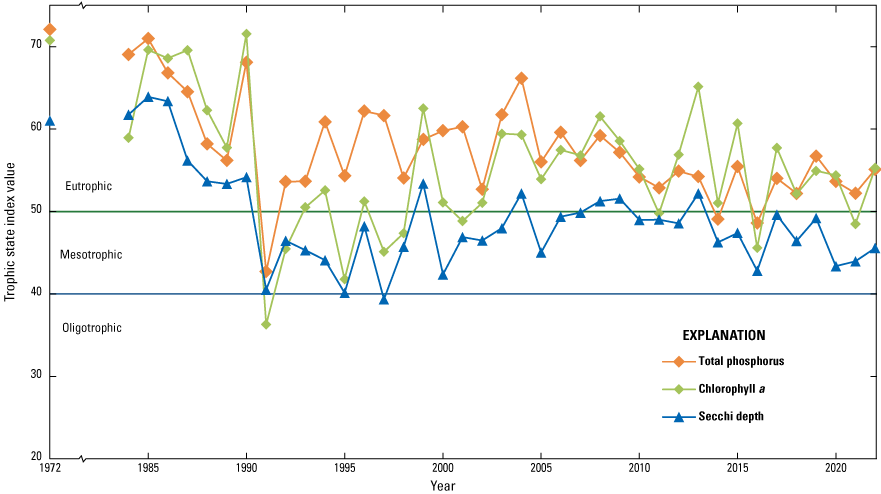
Annual summer mean trophic state index values for Delavan Lake, Wisconsin, based on summer mean near-surface total phosphorus and chlorophyll a concentrations, and Secchi depths, 1972–2022.
Water and Phosphorus Loading to Delavan Lake
During WYs 1984–2021, total annual precipitation near Delavan Lake ranged from 0.562 m in WY 2021 to 1.259 m in WY 2008 (table 2). The long-term mean annual precipitation during WYs 1984–2021 was 0.948 meter per year (m/yr). During WYs 2017–21, mean annual precipitation was 1.003 m/yr, which is about 6 percent more than the long-term mean.
Table 2.
Annual input (loads) of water and phosphorus to Delavan Lake, Wisconsin, by source, for water years 1984–2021.[HWY50, Highway 50; USGS, U.S. Geological Survey; NM, not measured]
Evaporation from the lake surface was assumed to be a constant value of 0.902 m/yr (Farnsworth and Thompson, 1982). Therefore, the long-term mean annual amount of water added by precipitation minus evaporation during WYs 1984–2021 was 0.322×106 cubic meters per year (m3/yr), and the mean annual input from precipitation minus evaporation during WYs 2017–21 was 0.708×106 m3/yr (table 2). Therefore, although precipitation was only about 6 percent more than the long-term mean, precipitation minus evaporation was estimated to be about twice the long-term mean.
Daily inflow from Jackson Creek, the main tributary to the lake, was measured or estimated at Highway 50 (see “Stream Monitoring” section; fig. 6A; table 2). The mean annual flow at Highway 50 was 16.0×106 m3/yr (17.9 cubic feet per second [ft3/s]) during WYs 1984–2021 and was 29.0×106 m3/yr (32.5 ft3/s) during WYs 2017–21. Assuming that the flows estimated at Highway 50 and the unmonitored areas in WYs 1984–85 by Field and Duerk (1988) remained at a similar proportion, the mean annual flow from the remaining unmonitored areas during WYs 2017–21 would be 12.9×106 m3/yr (14.4 ft3/s), and the total flow from the entire drainage basin during WYs 2017–21 would be 42.6×106 m3/yr (47.7 ft3/s). Mean annual flows to the lake increased during WYs 2017–21 from flows during WYs 1984–2015; mean annual flows to the lake were almost twice as high as the long-term mean (table 2).
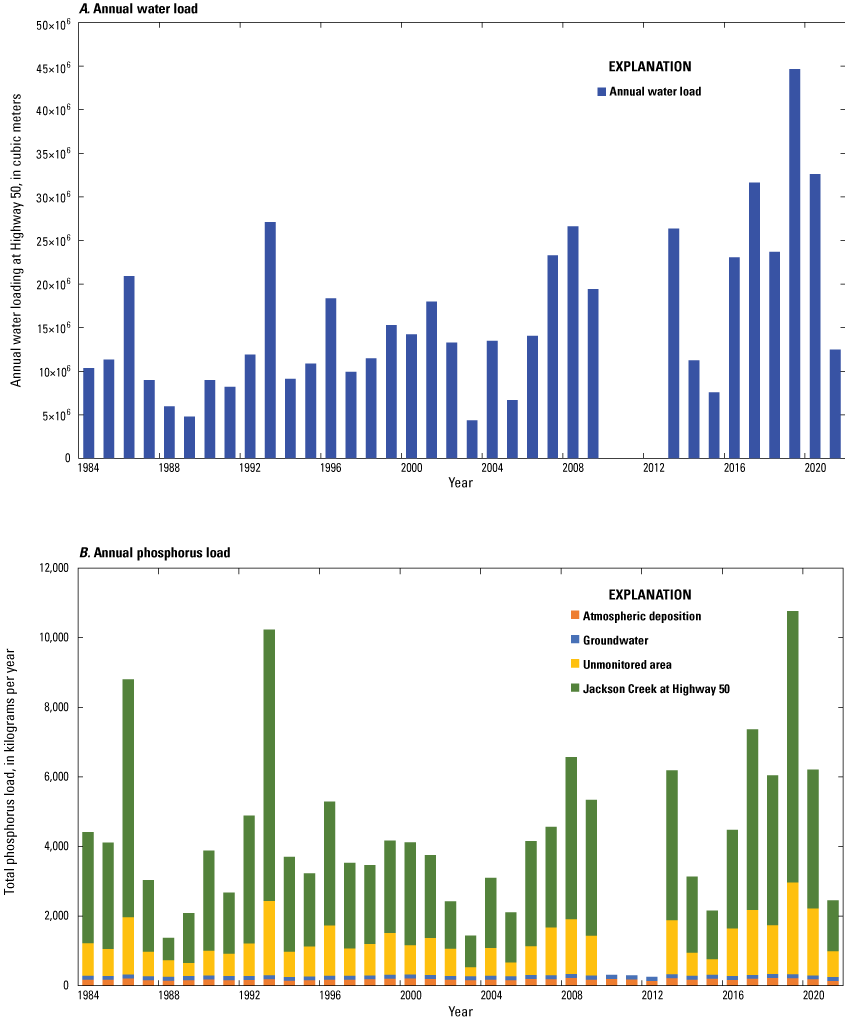
(A) Annual water input (loads) to Delavan Lake from Jackson Creek and (B) annual phosphorus input (loading) to Delavan Lake by source for water years 1984–2021. Annual water loads and phosphorus loads from Jackson Creek and the unmonitored areas were not measured in water years 2010–12.
The long-term mean annual loading for water to Delavan Lake (WYs 1984–2021) was 23.5×106 m3/yr, and mean annual loading during WYs 2017–21 was 42.6×106 m3/yr. Therefore, the long-term mean residence time of water in the lake was 2.26 years but was 1.24 years during WYs 2017–21. The mean annual precipitation, runoff, total water input to the lake, and residence time during the base period are different from their long-term means but may emulate anticipated future hydrologic conditions caused by the effect of climate change expected in the future for the Delavan Lake area (Katt-Reinders and Pomplun, 2011).
The estimated flows (summarized in table 2) and measured TP concentrations at Highway 50 were used to estimate annual phosphorus loads to the lake. Total annual phosphorus loads at Highway 50 (table 2) were estimated with integration methods (Porterfield, 1972; Koltun and others, 2006). The mean annual phosphorus load at Highway 50 during WYs 1984–2021 was 3,090 kilograms per year (kg/yr), but annual loads ranged from 636 kg/yr in WY 1988 to 7,800 kg/yr in WY 2019 (table 2; fig. 6B). The mean annual phosphorus load during WYs 2017–21 was 4,550 kg/yr, which is about 47 percent more than the long-term mean (3,090 kg/yr; table 2).
The estimated annual phosphorus loads and flows at Highway 50 were used to determine annual volumetrically weighted mean TP concentrations (annual TP load divided by annual flow; table 2). The annual volumetrically weighted mean TP concentrations at Highway 50 decreased from about 0.27 mg/L in WYs 1984–93 to about 0.15 mg/L in WYs 2017–21 (fig. 7). This is about a 43-percent decrease in TP concentrations. This decrease in TP concentrations was probably the result of the earlier decreases in point-source loads in the basin (Robertson and others, 2000) and efforts in the basin to reduce nonpoint phosphorus export from its drainage basin.
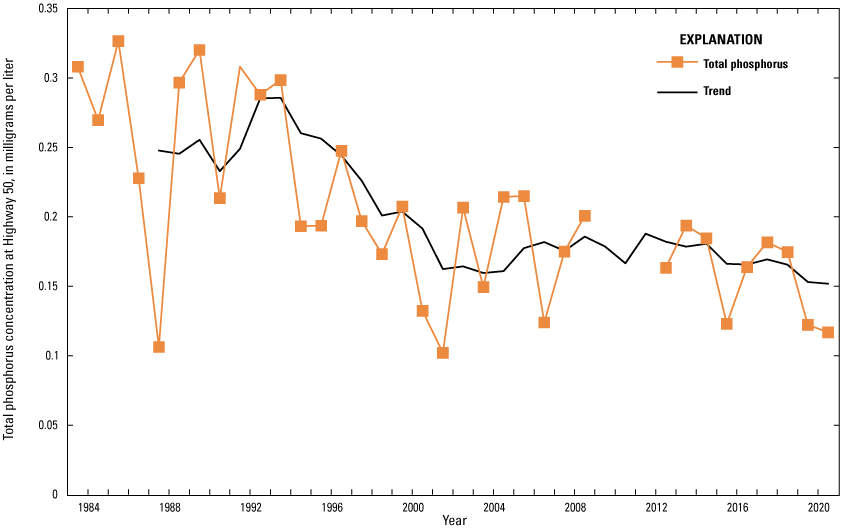
Annual volumetrically weighted mean total phosphorus concentrations at Jackson Creek at Highway 50 (U.S. Geological Survey station 05431017) for water years 1984–2021. The 5-year moving average (trend), plotted in year 3, is shown on the plot.
In addition to the criteria for TP impairment established for lakes, there are TP concentration criteria for the streams and rivers in Wisconsin. The TP concentration criterion for impairment in wadeable streams, like Jackson Creek, is for the median TP concentration from May through October not to exceed 0.075 mg/L (Wisconsin Department of Natural Resources, 2019). Based on the daily loads and flows measured at Highway 50, mean daily TP concentrations were computed. The median May through October TP concentration has decreased from 0.269 mg/L during 1984–88 to 0.168 mg/L during 2017–21, which is still much higher than the 0.075-mg/L criterion for TP impairment. Therefore, a reduction in loading of 55 percent from the 2017–21 base period is still needed for Jackson Creek not to be considered impaired for high TP concentrations.
To estimate the phosphorus load from the unmonitored areas during WYs 1984–2021, different methods were used. During WYs 1984–85, annual loads were obtained from Field and Duerk (1988). For WYs 1986–99, the estimated flows for the unmonitored areas (table 2) were multiplied by the mean volumetrically weighted concentration during WYs 1984–85 (0.177 mg/L; Field and Duerk, 1988). For WYs 2000–21, the estimated flows for the unmonitored areas were multiplied by the mean volumetrically weighted concentration from WYs 1984–85, reduced by 25 percent (0.133 mg/L) to account for changes in management in its drainage basin. The mean annual phosphorus load from the unmonitored areas was estimated to be 1,050 kg/yr during WYs 1984–2021 and was estimated to be 1,710 kg/yr during WYs 2017–21. Therefore, the total annual loading from the drainage basin of Delavan Lake (phosphorus load at Jackson Creek at Highway 50 plus that from the unmonitored areas) during WYs 2017–21 was estimated to be 6,260 kg/yr.
Field and Duerk (1988) estimated the total annual load of phosphorus from shallow wells installed around the shoreline of Delavan Lake that were monitored on a quarterly basis during 1983–85. The annual phosphorus loads from groundwater over the entire period of this study were assumed to be the same as that estimated by Field and Duerk (1988; 115 kg/yr).
Atmospheric phosphorus input to Delavan Lake was estimated from phosphorus dryfall deposition rates measured during nonprecipitation periods and TP concentrations measured in wetfall (rain and snow) at a station installed near the DLSD office during 2005–9 (Robertson and Kennedy, 2021; fig. 1). Phosphorus dryfall deposition rates were measured for 16 periods without precipitation. The dryfall deposition rates had distinct seasonality; the lowest deposition rates were during December through February (usually less than 0.0002 kilogram per hectare per day), and the highest deposition rates were in spring and late fall (usually more than 0.0004 kilogram per hectare per day). The individual deposition rates were used to estimate mean monthly dryfall deposition rates (fig. 8) and a total annual dryfall deposition rate (0.117 kilogram per hectare per year [(kg/ha)/yr]; table 3) or 81.6 kg/yr on the surface of Delavan Lake. Annual dryfall deposition of phosphorus on Delavan Lake was assumed to be constant at 81.6 kg/yr.
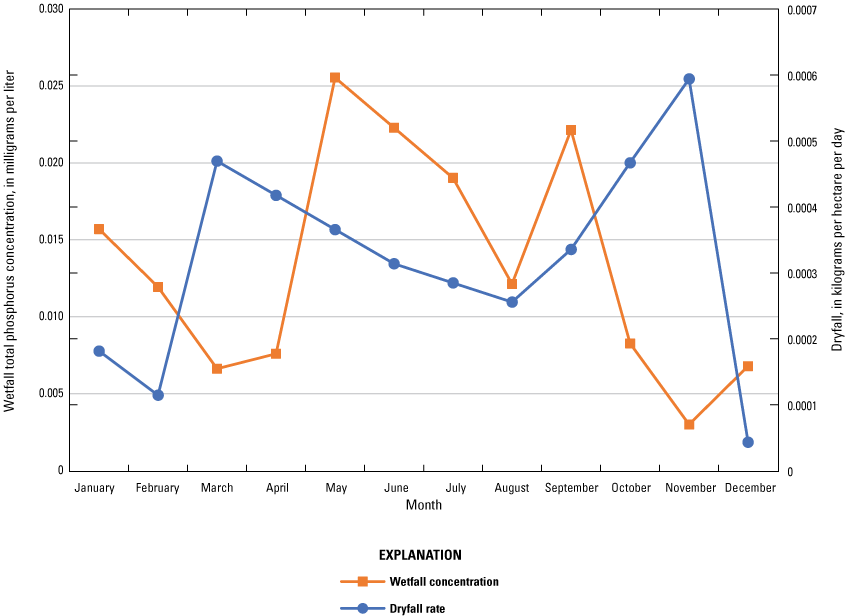
Mean monthly total phosphorus concentrations in wetfall and phosphorus deposition rates in dryfall near Delavan Lake, Wisconsin, based on data collected during 2005–9. Wetfall concentrations were adjusted to remove dryfall deposition during its collection period.
Table 3.
Mean monthly wetfall total phosphorus concentrations and total monthly and annual phosphorus deposition rates (dryfall) for southern Wisconsin. Data were collected near Delavan Lake, Wisconsin. Wetfall concentrations were adjusted to remove input from dryfall during its collection period. Monthly precipitation was measured at Geneva and Clinton, Wisconsin.[mg/L, milligram per liter; mm, millimeter; (kg/ha)/yr, kilogram per hectare per year]
TP concentrations in wetfall, each adjusted for dryfall during the wetfall-collection period, measured in 40 precipitation events during 2005–9 were averaged by month. TP concentrations in precipitation were generally lowest in winter and spring (about 0.010 mg/L) and highest in early and late summer (about 0.025 mg/L; fig. 8). A mean annual wetfall concentration (0.0147 mg/L) was computed by weighting the monthly mean TP concentrations by the respective long-term (1983–2019) mean monthly precipitation near Delavan (measured at Geneva, Wis., and Clinton, Wis.; table 3). Monthly inputs of phosphorus from precipitation (wetfall) on Delavan Lake were then computed by multiplying the total monthly precipitation measured near Delavan Lake, the area of the lake, and the mean monthly TP concentrations in table 3. The mean annual phosphorus input from precipitation (wetfall) was 96.8 kg/yr during WYs 1984–21 and was 110 kg/yr during WYs 2017–21. Therefore, the long-term mean annual atmospheric phosphorus input was 178 kg/yr during WYs 1984–21 and was 191 kg/yr during WYs 2017–21 (table 2).
The mean annual external phosphorus input to Delavan Lake was estimated to be 4,430 kg/yr during WYs 1984–2021 and was estimated to be 6,570 kg/yr during WYs 2017–21 (table 2; fig. 6). The mean annual external phosphorus input during WYs 1989–98 (the reference years for the Rock River TMDL) was 4,300 kg/yr, which is close to the long-term mean annual phosphorus input to the lake. Annual external phosphorus inputs ranged from 1,370 kg/yr in WY 1988 to 10,800 kg/yr in WY 2019. Tributary inputs, including inputs from unmonitored areas, were the dominant source of phosphorus to Delavan Lake (during WYs 1984–2021, the drainage basin contributed 4,140 kg/yr or 93.4 percent of total external inputs; during WYs 2017–21, the drainage basin contributed 6,260 kg/yr or 95.3 percent of total external inputs). During WYs 2017–21, atmospheric input (combined wetfall and dryfall) and groundwater, collectively referred to as “uncontrollable phosphorus sources,” contributed about 5 percent of the external phosphorus loading. The mean annual external phosphorus input to Delavan Lake during WYs 2017–21 was higher than the long-term mean annual input but may represent new hydrologic conditions for the Delavan Lake area (Katt-Reinders and Pomplun, 2011) or represent hydrologic conditions for a possible sequence of years that may be detected more frequently in the future.
Response in Near-Surface Water Quality to Changes in Phosphorus Loading
To estimate how the near-surface water quality (TP and Chl a concentrations and SDs) in Delavan Lake is expected to respond to changes in phosphorus loading, 18 scenarios were simulated with the Canfield-Bachmann TP models (eqs. 4 and 5; Canfield and Bachmann, 1981), the Carlson (1977) TSI relations (eqs. 1–3), and the Jones and Bachmann (1976) TP–Chl a relation (eq. 6). Scenario 1 simulated the mean conditions for the base period of WYs 2017–21, and the results of this scenario were used to determine if and how the output from all scenarios could be adjusted (calibrated) to better estimate conditions in Delavan Lake. Because of the relatively long residence time of water in the lake (longer than 1 year), individual year-by-year simulations were not completed. Model results were adjusted (in other words, calibrated) based on the percentage difference between the results for scenario 1 (without adjustment) and the mean water quality measured in the lake during WYs 2017–21. An additional 17 simulations (scenarios 2–18) were then completed to estimate the response in water quality to basin-wide changes in potentially controllable phosphorus sources including all external sources except inputs from groundwater and atmospheric deposition: decreases in the potentially controllable phosphorus sources by 90, 80, 70, 60, 50, 35, 30, 21, 20, and 10 percent and increases in potentially controllable phosphorus sources by 10, 20, 30, 50, 75, and 100 percent. By not including internal loading in the models in any of the scenarios, it was assumed that the amount of phosphorus from internal sediment recycling would change at a percentage similar to the change in potentially controllable external phosphorus sources. Internal sediment recycling is not expected to change immediately but is expected to eventually come to equilibrium with the new external phosphorus loading with a change directly proportional to the percentage change in all external sources (Robertson and others, 2018; Robertson and Diebel, 2020).
Four types of data were required as input into the empirical eutrophication models: measured water-quality data (table 1), morphometric data (described in the text), hydrologic data (table 2), and phosphorus-loading data (table 2). The specific inputs used in the models or used to estimate parameters used in the models are summarized in table 4. Although annual phosphorus-loading data are typically used as inputs into the models, the models simulate water quality during the summer season; therefore, the geometric mean water-quality data for summer in table 4 were used to validate and adjust (calibrate) the models as explained below.
Table 4.
Summary of inputs to the eutrophication models used for Delavan Lake. Water-quality data are based on the 2017–21 base period.[m2, square meter; m3, cubic meter; m3/yr, cubic meter per year; m, meter; kg/yr, kilogram per year; mg/L, milligram per liter; µg/L, microgram per liter]
Verification and Adjustments for Model Biases
To determine how well the simulated TP concentrations from the Canfield-Bachmann natural-lake and artificial-lake models (eqs. 4 and 5) are expected to simulate changes in TP concentrations in Delavan Lake, the models were used to simulate the near-surface TP concentration using the mean annual external phosphorus loading for the base period of WYs 2017–21. The mean simulated TP concentration was 0.050 mg/L (table 5), which is higher than the geometric mean of the TP concentrations measured in the lake (0.030 mg/L). Therefore, all simulated TP concentrations were decreased by 40.0 percent (bias adjusted). Part of the discrepancy in the measured and unbiased-adjusted simulated TP concentrations may have been caused by short circuiting of water and phosphorus from the Jackson Creek inlet to the outlet that resulted in the estimated load being greater than the load actually affecting the lake. There may be considerable short circuiting of the phosphorus through Delavan Lake considering the proximity of major inlet and the outlet of the lake. Therefore, this adjustment for model bias is required to use the results of the Canfield-Bachmann models.
Table 5.
Simulated response of near-surface total phosphorus in Delavan Lake, Wisconsin, to various phosphorus-loading scenarios based on the Canfield-Bachmann natural-lake and artificial-lake models (Canfield and Bachmann, 1981). Trophic state index values were computed using equation 1 (Carlson, 1977). Total phosphorus loading does not include inputs from internal sediment recycling. All changes in loading are based on percentage changes in potentially controllable phosphorus loading (all but atmospheric and groundwater input). Surface area was 6,964,000 square meters, volume was 52,960,000 cubic meters, mean depth was 7.61 meters, and residence time was 1.24 years.[TP, total phosphorus concentration; TSI, trophic state index values; %, percent]
During the 2017–21 base period, the mean TSIChl a was 0.003 times higher than the mean TSITP (TSIChl a=TSITP×1.003) and the mean TSISD was 0.859 times the mean TSITP (TSISD=TSITP×0.859). To determine how well the Carlson TSI relation approach is expected to simulate Chl a concentrations and SDs, the bias-adjusted TP concentration converted to a TSI value (TSITP) was first multiplied (adjusted) by the typical differences in the TSI values observed during WYs 2017–21; the adjusted TSIChl a value and adjusted TSISD value were then used to compute the Chl a concentration and SD with similar TSI values. Given a TSITP value of 53.1 (from the bias-adjusted Canfield-Bachmann models, table 5) and TSIChl a of 53.2 (from the typical TSI relation), the simulated Chl a concentration was 10.1 µg/L (from eq. 2), whereas the measured geometric mean value was 8.9 µg/L. Therefore, the TSI approach seems to slightly overestimate Chl a concentrations. To remove the bias in use of the TSI approach, all simulated Chl a concentrations were decreased by 11.1 percent. Given a TSITP value of 53.1 and TSISD of 45.6, the simulated SD was 2.77 m (eq. 3), whereas the measured geometric mean value was 2.38 m. Therefore, the TSI approach seems to overestimate SDs. To remove the biases in the use of the TSI approach, all simulated SDs were decreased by 12.2 percent.
To determine how well the Jones and Bachmann (1976) TP–Chl a relation is expected to simulate mean Chl a concentrations, the measured mean summer TP concentration for WYs 2017–21 was used to simulate the mean summer Chl a concentration. Given the measured TP concentration of 0.030 mg/L, the simulated Chl a concentration from the TP–Chl a relation was 11.5 µg/L, whereas the measured geometric mean concentration was 8.9 µg/L. Therefore, this relation seemed to overestimate Chl a concentrations. To remove the potential bias in this relation, all simulated Chl a concentrations using the Jones and Bachman TP–Chl a relation were decreased by 22.6 percent.
Simulated Response in Total Phosphorus, Chlorophyll a, and Secchi Depth
In this section of the report, the simulated responses of near-surface TP and Chl a concentrations and SDs to changes in external phosphorus loading from the Delavan Lake drainage basin are described.
Total Phosphorus
Based on simulations representing a range of basinwide changes in loading from potentially controllable external phosphorus sources (scenarios 2–18) with the Canfield-Bachmann models, changes in mean June–September TP concentrations are simulated to be smaller, on a percentage basis, than the changes in external phosphorus loadings (table 5; fig. 9A). Furthermore, the responses to reductions in phosphorus loading are expected to be larger on a percentage basis than similar increases in loading. Changes in TP concentrations were about 53–83 percent of the changes in external phosphorus loading. For example, a 70-percent decrease in the potentially controllable external phosphorus sources, which equates to a 67-percent decrease in total external phosphorus loading, is expected to result in a 53-percent decrease in TP concentrations, whereas a 70-percent increase in potentially controllable phosphorus sources is expected to result in a 38-percent increase in TP concentrations. Because the current (WYs 2017–21) geometric mean TP concentration was 0.030 mg/L, no change in loading is needed for the geometric mean TP concentration in the lake to reach the 0.030-mg/L goal during June–September (the WDNR criterion for TP impairment for Delavan Lake).
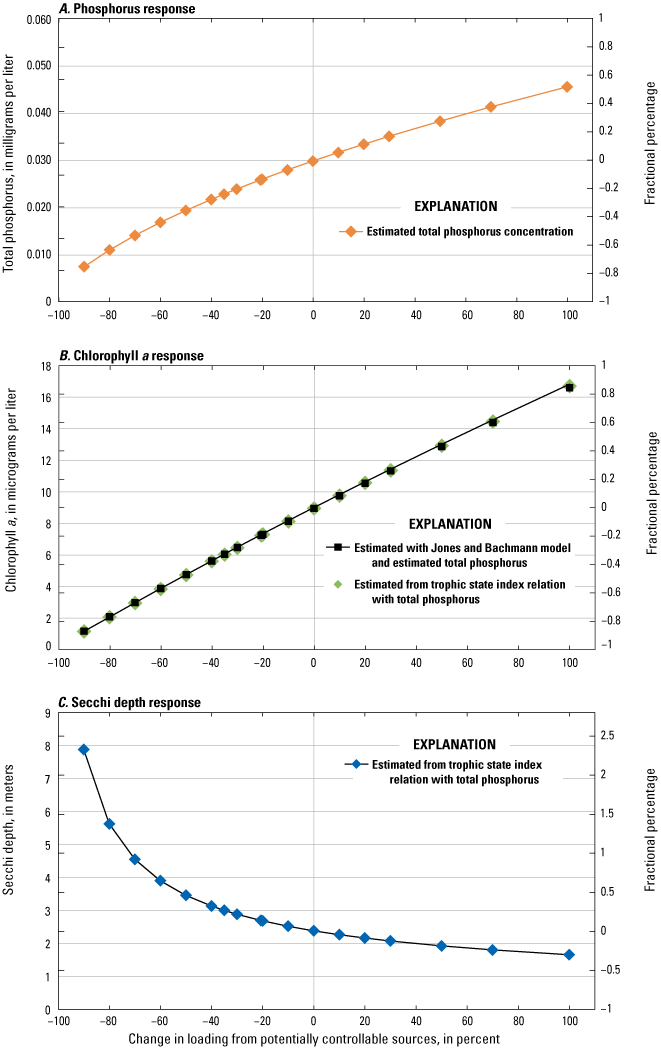
Simulated changes in mean summer water quality in Delavan Lake, Wisconsin, in response to various phosphorus-loading scenarios simulated using the Canfield-Bachmann natural-lake and artificial-lake models (Canfield and Bachmann, 1981), the Carlson (1977) trophic state index relations, and the Jones and Bachmann (1976) total phosphorus–chlorophyll a relation for (A) total phosphorus (June 1–September 15), (B) chlorophyll a (July 15–September 15), and (C) Secchi depth (July 15–September 15).
The WYs 2017–21 base period included prolonged periods of heavier than normal precipitation, runoff, and higher than normal phosphorus loading to the lake. During WYs 2013–17, the mean annual phosphorus loading to the lake was 3,990 kg/yr, and the measured geometric mean TP concentration in the lake during June–September was 0.028 mg/L. Therefore, if the lake experiences typical hydrology in the future or the hydrology experienced during the WYs 2017–21 base period, no reduction in phosphorus loading may be needed to maintain the 0.030-mg/L criterion for TP impairment. However, if precipitation increases more than what was experienced during the base period, a reduction in phosphorus loading may be needed.
Chlorophyll a
Two approaches (the Carlson TSI approach and Jones and Bachmann TP–Chl a relation approach) were used to determine how near-surface mean July–September Chl a concentrations are expected to respond to changes in phosphorus loading and the expected changes in TP concentrations simulated with the Canfield-Bachmann models (fig. 9B; table 6). The response in Chl a concentrations predicted with the typical difference in TSI values (TSIChl a=TSITP×1.003) adjusted for the small bias simulated for WYs 2017–21 had a linear response to changes in potentially controllable external phosphorus loading, and the percentage changes were similar to the percentage change in total external phosphorus loading. The response in near-surface mean July–September Chl a concentrations also was estimated with the Jones and Bachmann TP–Chl a relation (eq. 6). The response in mean Chl a concentrations to changes in potentially controllable phosphorus sources adjusted for the small bias simulated for WYs 2017–21 was similar to that of the Carlson (1977) TSI approach.
Table 6.
Simulated response of near-surface mean summer (July–September) chlorophyll a concentrations and Secchi depths in Delavan Lake, Wisconsin, to various phosphorus-loading scenarios based on the Canfield-Bachmann natural-lake and artificial-lake models (Canfield and Bachmann, 1981), Carlson (1977) trophic state index equations, and Jones and Bachmann (1976) total phosphorus–chlorophyll a relation. Total phosphorus loading does not include inputs from internal sediment recycling. All changes in loading are based on percentage changes in controllable phosphorus loading (all but atmospheric and groundwater input). Surface area was 6,964,000 square meters, volume was 52,960,000 cubic meters, mean depth was 7.61 meters, and residence time was 1.24 years.[TP, total phosphorus; TSI, trophic state index; Chl a, chlorophyll a; SD, Secchi depth; %, percent]
Based on the variability in Chl a concentrations observed in Delavan Lake during WYs 2017–21 (standard deviation of 0.62 natural logarithmic unit), the lake would be required to reduce its geometric mean Chl a concentration from 8.9 to 7.2 μg/L (a 19-percent reduction in all measured values) for Chl a concentrations greater than 20 μg/L to be detected no more than 5.0 percent of the time. Based on these two approaches, a 21-percent reduction in loading from the potentially controllable external phosphorus sources (reduction in total external phosphorus loading from 6,570 to 5,270 kg/yr) is simulated to be needed to cause the geometric mean Chl a concentration to decrease from 8.9 µg/L to the goal of 7.2 µg/L and result in Chl a concentrations greater than 20 μg/L being detected no more than 5.0 percent of the time.
Secchi Depth
The Carlson (1977) TSI relation approach was used to determine the expected response in the geometric mean of the SDs during July–September to changes in phosphorus loading and the changes in TP concentrations simulated with the Canfield-Bachmann models. Based on the simulated TP concentrations and the relation in TSI values (TSISD=TSITP×0.859), SDs are expected to have a nonlinear response to changes in potentially controllable external phosphorus sources (fig. 9C; table 6). For example, a 70-percent decrease in the potentially controllable external phosphorus sources is expected to cause a 91-percent increase in SDs, whereas a 70-percent increase in the potentially controllable sources is expected to cause only a 24-percent decrease in SDs. A 35-percent reduction in loading from potentially controllable phosphorus sources (reduction in total external loading from 6,570 to 4,380 kg/yr) is expected to cause the mean July–September SDs to increase from 2.4 m to the 3.0-m goal for the lake. Given that the geometric mean of the SDs during July–August for the 2017–21 base period (2.41 m) was greater than that for July–September (2.38 m), the phosphorus-load reduction needed to increase the summer mean water clarity to 3.0 m also should improve the mean July–August SD to be greater than 3.0 m.
Although the precipitation, runoff, total water input to the lake, and residence time during the base period of WYs 2017–21 were different from the long-term means (WYs 1984–21), the 2017–21 weather conditions may approximate the anticipated future conditions as affected by climatic change. Therefore, the reductions in phosphorus loading needed to reach the Chl a and SD goals should be reasonable for lake management planning initiatives.
Summary and Conclusions
In the mid-1800s, Delavan Lake was a beautiful, pristine lake with excellent water quality and a good fishery. As a result of increased phosphorus inputs from urban and agricultural activities in its drainage basin, the lake’s water quality in the 1970s was considered one of the worst in Wisconsin. Because of the poor water quality, an extensive rehabilitation effort was completed during 1989–92 to reduce total phosphorus (TP) and chlorophyll a (Chl a) concentrations, improve water clarity (Secchi depth [SD]), and improve the lake’s fishery. The rehabilitation effort was initially successful, and the lake was classified as oligotrophic based on TP, Chl a, and SD, but because of high phosphorus loading to the lake, TP concentrations quickly increased to greater than the 0.034-milligram per liter (mg/L) goal established in the 1986 rehabilitation plan. However, the Chl a concentrations and SDs remained better than their original goals for the lake (mean summer Chl a concentration less than 14 micrograms per liter [μg/L] and mean summer SD greater than 1.5 meters [m]) because of food-web manipulations. Continued management in the Delavan Lake drainage basin (detention pond and inlet dredging) likely resulted in a gradual decrease in the TP concentrations to below the original goal for the lake.
Although the water quality in Delavan Lake was better than the goals defined in the 1986 rehabilitation plan, in 2016, Delavan Lake was listed as impaired for excessive algal growth (high Chl a concentrations) by the Wisconsin Department of Natural Resources (WDNR) and high phosphorus input was listed at its likely cause. Recent (2017–21) near-surface mean summer TP concentrations in the lake were at the WDNR criterion for TP impairment (0.030 mg/L) for a deep drainage lake, but the mean summer water clarity (SD) was shallower than the 3.0-m goal set by the community. Therefore, the primary goals of this report were to describe changes in in-lake TP and Chl a concentrations and SDs, and phosphorus loading to Delavan Lake (since 1972 for lake water quality and since 1984 for phosphorus loading); define current (WYs 2017–21) in-lake TP and Chl a concentrations, and SDs and phosphorus loading; develop response curves for TP and Chl a concentrations, and SD to changes in external phosphorus loading to the lake; and determine the magnitude in phosphorus-load reductions (compared to the WYs 2017–21 base period) needed to reach the new goals set for the lake.
During the 2017–21 base period, the geometric means for their respective summer evaluation periods were 0.030 mg/L for TP concentration, 8.9 μg/L for Chl a concentration (corresponding to Chl a concentrations greater than 20 μg/L occurring on about 9.6 percent of the days), and 2.4 m for SD. The geometric mean Chl a concentration and SD were worse than their respective goals or criteria for impairment, and a reduction in phosphorus loading to the lake is needed to reach their goals. During WYs 2017–21, the mean annual phosphorus loading to the lake was 6,570 kilograms per year (kg/yr), and 306 kg/yr came from uncontrollable sources (atmospheric deposition and groundwater). Mean annual loading during WYs 2017–21 was about 48 percent higher than the long-term (WYs 1984–21) loading.
A combination of empirical eutrophication models was used to estimate the response in TP and Chl a concentrations and SDs to changes in phosphorus loading from its drainage basin and used to determine the reduction in phosphorus loading for the lake to reach its water-quality goals. Based on the Canfield-Bachmann simulated TP concentrations, Carlson trophic state index relations, and the Jones and Bachmann TP–Chl a relation, a 21-percent reduction in loading from potentially controllable external phosphorus sources from those of the base period (6,570 to 5,270 kg/yr) would likely result in the geometric mean Chl a concentration to decrease to 7.2 µg/L and result in Chl a concentrations greater than 20 μg/L being detected no more than 5.0 percent of the time during July–September. Based on the Canfield-Bachmann simulated TP concentrations and Carlson trophic state index relation approach, a 35-percent reduction in loading from potentially controllable phosphorus sources from those of the base period (6,570 to 4,380 kg/yr) would likely result in mean July–September and July–August SDs to increase from 2.4 to 3.0 m. Therefore, for Delavan Lake to reach the current water-quality criteria for impairment and goals for all three constituents, a 35-percent reduction loading from potentially controllable phosphorus sources when compared to the 2017–21 base period used in this study would be necessary, which equates to a reduction in TP loading from 6,570 to 4,380 kg/yr. The base period of WYs 2017–21 had greater than normal precipitation, runoff, and phosphorus loading to the lake when compared to WYs 1984–2015. Therefore, part of this reduction would be achieved if typical hydrologic conditions existed, such as during WYs 2013–17 when the mean summer TP concentration was less than the WDNR criterion for TP impairment. However, given that the conditions during the base period emulate anticipated future conditions as affected by climatic change, the 35-percent phosphorus-load reduction should provide a reasonable goal for lake management planning initiatives.
As part of the Rock River total maximum daily load (TMDL), a 49-percent reduction in phosphorus loading for the area near Delavan Lake was found to be needed to likely improve the water quality of the Rock River and its tributaries. The hydrology for the reference years used in the TMDL was close to the long-term average; therefore, the 35-percent reduction in phosphorus loading to Delavan Lake to improve its water quality is less than that required as part of that TMDL. In addition to the criteria for TP impairment established for lakes, there is a water-quality criterion for TP impairment for the streams in Wisconsin (for the median TP concentration from May through October not to exceed 0.075 mg/L). The median May through October TP concentration for Jackson Creek at Highway 50 (U.S. Geological Survey station 05431017) during 2017–21 was 0.168 mg/L. Therefore, a reduction in phosphorus loading of 55 percent from that during the 2017–21 base period is needed for Jackson Creek not to be considered impaired for high TP concentrations.
The response curves created in this study can be used to develop load-reduction goals for the Delavan Lake drainage basin. These load-reduction goals can be incorporated into management plans for the lake that, if followed, could improve the water quality in the lake, remove Delavan Lake from being considered impaired for high chlorophyll a concentrations, and reach the water-clarity goals set for the lake.
References Cited
Berrini & Associates, LLC, and Northwater Consulting, 2016, Delavan Lake Watershed Implementation Plan: Town of Delavan, Walworth County, Wisconsin, prepared by Berrini & Associates, LLC, and Northwater Consulting, 163 p., accessed December 1, 2022, at https://www.co.walworth.wi.us/DocumentCenter/View/3284/Delavan-Lake-WIP-Final-2-2-16.
Cadmus Group, 2011, Total maximum daily loads for total phosphorus and total suspended solids in the Rock River Basin: U.S. Environmental Protection Agency and Wisconsin Department of Natural Resources, prepared by Cadmus Group, 210 p., accessed December 1, 2022, at https://dnr.wisconsin.gov/topic/TMDLs/RockRiver/index.html.
Canfield, D.E., Jr., and Bachmann, R.W., 1981, Prediction of total phosphorus concentrations, chlorophyll a, and Secchi depths in natural and artificial lakes: Canadian Journal of Fisheries and Aquatic Sciences, v. 38, no. 4, p. 414–423. [Also available at https://doi.org/10.1139/f81-058.]
Carlson, R.E., 1977, A trophic state index for lakes: Limnology and Oceanography, v. 22, no. 2, p. 361–369. [Also available at https://doi.org/10.4319/lo.1977.22.2.0361.]
Farnsworth, R.K., and Thompson, E.S., 1982, Mean monthly, seasonal, and annual pan evaporation for the United States: Washington, D.C., National Oceanic and Atmospheric Administration, National Weather Service, NOAA Technical Report NWS 34, 82 p. [Also available at https://www.weather.gov/media/owp/oh/hdsc/docs/TR34.pdf.]
Field, S.J., and Duerk, M.D., 1988, Hydrology and water quality of Delavan Lake in southeastern Wisconsin: U.S. Geological Survey Water-Resources Investigations Report 87–4168, 107 p. [Also available at https://doi.org/10.3133/wri874168.]
Katt-Reinders, E., and Pomplun, S., 2011, Wisconsin’s changing climate—Impacts and adaptation: Madison, Wis., Wisconsin Initiative on Climate Change Impacts, 211 p., accessed December 1, 2022, at https://wicci.wisc.edu/2011-assessment-report/.
Koltun, G.F., Eberle, M., Gray, J.R., and Glysson, G.D., 2006, User’s manual for the Graphical Constituent Loading Analysis System (GCLAS): U.S. Geological Survey Techniques and Methods, book 4, chap. C1, 51 p. [Also available at https://doi.org/10.3133/tm4C1.]
Lathrop, R.C., 1992, Lake Mendota and the Yahara River chain, chap. 3 of Kitchell, J.F., ed., Food web management—A case study of Lake Mendota: New York, Springer-Verlag, p. 17–29. [Also available at https://doi.org/10.1007/978-1-4612-4410-3_3.]
Manteufel, S.B., Olson, D.L., Robertson, D.M., and Goddard, G.L., 2012, Water-quality and lake-stage data for Wisconsin lakes, water years 2008–2011: U.S. Geological Survey Open-File Report 2012–1238, 554 p, accessed December 1, 2022, at https://doi.org/10.3133/ofr20121238.
Midwestern Regional Climate Center, 2022, cli-MATE: Midwestern Regional Climate Center database, accessed December 1, 2022, at https://mrcc.purdue.edu/CLIMATE/.
Porterfield, G., 1972, Computation of fluvial-sediment discharge: U.S. Geological Survey Techniques of Water-Resources Investigations, book 3, chap. C3, 66 p. [Also available at https://doi.org/10.3133/twri03C3.]
Robertson, D.M., and Diebel, M.W., 2020, Importance of accurately quantifying internal loading in developing phosphorus reduction strategies for a chain of shallow lakes: Lake and Reservoir Management, v. 36, no. 4, p. 391–411. [Also available at https://doi.org/10.1080/10402381.2020.1783727.]
Robertson, D.M., Goddard, G.L., Helsel, D.R., and MacKinnon, K.L., 2000, Rehabilitation of Delavan Lake, Wisconsin: Lake and Reservoir Management, v. 16, no. 3, p. 155–176. [Also available at https://doi.org/10.1080/07438140009353961.]
Robertson, D.M., Juckem, P.F., Dantoin, E.D., and Winslow, L.A., 2018, Effects of water level and climate on the hydrodynamics and water quality of Anvil Lake, Wisconsin, a shallow seepage lake: Lake and Reservoir Management, v. 34, no. 3, p. 211–231. [Also available at https://doi.org/10.1080/10402381.2017.1412374.]
Robertson, D.M., and Kennedy, J.L., 2021, Eutrophication models to simulate changes in the water quality of Green Lake, Wisconsin in response to changes in phosphorus loading, with supporting water-quality data for the lake, its tributaries, and atmospheric deposition: U.S. Geological Survey data release, accessed December 1, 2022, at https://doi.org/10.5066/P9H85BK0.
Robertson, D.M., Rose, W.J., and Juckem, P.F., 2009, Water quality and hydrology of Whitefish (Bardon) Lake, Douglas County, Wisconsin, with special emphasis on responses of an oligotrophic seepage lake to changes in phosphorus loading and water level: U.S. Geological Survey Scientific Investigations Report 2009–5089, 41 p. [Also available at https://doi.org/10.3133/sir20095089.]
Robertson, D.M., Siebers, B.J., Ladwig, R., Hamilton, D.P., Reneau, P.C., McDonald, C.P., Prellwitz, S., and Lathrop, R.C., 2022, Response of Green Lake, Wisconsin, to changes in phosphorus loading, with special emphasis on near-surface total phosphorus concentrations and metalimnetic dissolved oxygen minima: U.S. Geological Survey Scientific Investigations Report 2022–5003, 77 p., accessed December 1, 2022, at https://doi.org/10.3133/sir20225003.
Sauer, V.B., 2002, Standards for the analysis and processing of surface-water data and information using electronic methods: U.S. Geological Survey Water-Resources Investigations Report 2001–4044, 91 p. [Also available at https://doi.org/10.3133/wri20014044.]
Schindler, D.W., Carpenter, S.R., Chapra, S.C., Hecky, R.E., and Orihel, D.M., 2016, Reducing phosphorus to curb lake eutrophication is a success: Environmental Science & Technology, v. 50, no. 17, p. 8923–8929. [Also available at https://doi.org/10.1021/acs.est.6b02204.]
University of Wisconsin-Stevens Point, 2022, Extension Lakes—CLMN training resources: University of Wisconsin-Stevens Point web page, accessed December 1, 2022, at https://www3.uwsp.edu/cnr-ap/UWEXLakes/Pages/programs/clmn/training.aspx#manuals.
U.S. Department of Agriculture, 2006, National Agriculture Imagery Program (NAIP) digital orthorectified images (DOQ), Wisconsin: U.S. Department of Agriculture, Natural Resources Conservation Service digital data, accessed December 1, 2020, at https://datagateway.nrcs.usda.gov/.
U.S. Geological Survey, 2018, US DH-59 depth integrating suspended hand line sampler, accessed December 1, 2022, at https://water.usgs.gov/fisp/products/4101005.html.
U.S. Geological Survey, 2022, USGS water data for the Nation: U.S. Geological Survey National Water Information System database, accessed December 1, 2022, at https://doi.org/10.5066/F7P55KJN.
Walker, W.W., 1996, Simplified procedures for eutrophication assessment and prediction—User manual: U.S. Army Corps of Engineers, Instruction Report W–96–2, [variously paged; 237 p.]. [Also available at https://apps.dtic.mil/sti/citations/ADA286912.]
Wisconsin Department of Natural Resources, 2020, Delavan Lake—Walworth County, Wisconsin DNR lake map: Wisconsin Department of Natural Resources, 3 sheets, scale 1:6,000, accessed December 1, 2022, at https://dnr.wi.gov/lakes/maps/DNR/0793600a.pdf.
Wisconsin Department of Natural Resources, 2016, Land cover data (Wiscland): Wisconsin Department of Natural Resources database, accessed December 1, 2022, at https://dnr.wisconsin.gov/maps/WISCLAND.
Wisconsin Department of Natural Resources, 2019, Wisconsin 2020 Consolidated Assessment and Listing Methodology (WisCALM)—Clean Water Act section 303(d) and 305(b) integrated reporting: Madison, Wis., Wisconsin Department of Natural Resources report, Guidance no. 3200–2019–04, 116 p., accessed December 1, 2022, at https://dnr.wisconsin.gov/topic/SurfaceWater/WisCALM.html.
Wisconsin Department of Natural Resources, 2021, Water condition lists—Impaired waters, restoration waters and healthy waters: Wisconsin Department of Natural Resources web page [previous lists], accessed December 1, 2022, https://dnr.wisconsin.gov/topic/SurfaceWater/ConditionLists.html.
Wisconsin Department of Natural Resources, 2022a, Citizen Lake Monitoring Network: Wisconsin Department of Natural Resources web page, accessed December 1, 2022, at https://dnr.wisconsin.gov/topic/lakes/clmn.
Wisconsin Department of Natural Resources, 2022b, Delavan Lake—Deep Hole: Wisconsin Department of Natural Resources web page, accessed December 1, 2022, at https://dnr.wi.gov/lakes/waterquality/Station.aspx?id=653215.
Wisconsin State Laboratory of Hygiene, 2020, Quality assurance manual (revision 18, June 2020): University of Wisconsin-Madison, Environmental Health Division, [variously paged; 188 p.]. [Also available at https://wwwtest.slh.wisc.edu/wp-content/uploads/2020/07/NELAC-QA-Manual-rev-18-2020-combined.pdf.]
Conversion Factors
International System of Units to U.S. customary units
Multiply | By | To obtain |
---|---|---|
centimeter (cm) | 0.3937 | inch (in.) |
millimeter (mm) | 0.03937 | inch (in.) |
meter (m) | 3.281 | foot (ft) |
kilometer (km) | 0.6214 | mile (mi) |
square meter (m2) | 0.0002471 | acre |
hectare (ha) | 2.471 | acre |
square kilometer (km2) | 247.1 | acre |
square meter (m2) | 10.76 | square foot (ft2) |
hectare (ha) | 0.003861 | square mile (mi2) |
square kilometer (km2) | 0.3861 | square mile (mi2) |
milliliter (mL) | 0.03381 | ounce, fluid (fl. oz) |
liter (L) | 33.81 | ounce, fluid (fl. oz) |
liter (L) | 0.2642 | gallon (gal) |
cubic meter (m3) | 264.2 | gallon (gal) |
cubic meter (m3) | 35.31 | cubic foot (ft3) |
cubic meter (m3) | 0.0008107 | acre-foot (acre-ft) |
cubic meter per year (m3/yr) | 0.000811 | acre-foot per year (acre-ft/yr) |
meter per year (m/yr) | 39.37 | inch per year (in/yr) |
kilogram per year (kg/yr) | 2.205 | pound avoirdupois per year (lb/yr) |
kilogram per hectare per day ([kg/ha]/d) | 0.8921 | pound per acre per year ([lb/acre]/d) |
kilogram per hectare per year ([kg/ha]/yr) | 0.8921 | pound per acre per year ([lb/acre]/yr) |
Supplemental Information
Concentrations of chemical constituents in water are given in either milligrams per liter (mg/L) or micrograms per liter (µg/L). Atmospheric deposition is given in kilograms per hectare per day ([kg/ha]/d).
A water year is the period from October 1 to September 30 and is designated by the year in which it ends; for example, water year 2021 was from October 1, 2020, to September 30, 2021.
Abbreviations
Chl a
chlorophyll a
DLSD
Delavan Lake Sanitary District
JCP
Jackson Creek at Petrie Road near Elkhorn
SD
Secchi depth
TMDL
total maximum daily load
TP
total phosphorus
TSI
trophic state index
TSIChl a
trophic state index value based on chlorophyll a
TSISD
trophic state index value based on Secchi depth
TSITP
trophic state index value based on total phosphorus
USGS
U.S. Geological Survey
WDNR
Wisconsin Department of Natural Resources
WY
water year
For more information about this publication, contact:
Director, USGS Upper Midwest Water Science Center
1 Gifford Pinchot Drive
Madison, WI 53726
608–828–9901
For additional information, visit: https://www.usgs.gov/centers/umid-water
Publishing support provided by the
Rolla Publishing Service Center
Disclaimers
Any use of trade, firm, or product names is for descriptive purposes only and does not imply endorsement by the U.S. Government.
Although this information product, for the most part, is in the public domain, it also may contain copyrighted materials as noted in the text. Permission to reproduce copyrighted items must be secured from the copyright owner.
Suggested Citation
Robertson, D.M., Siebers, B.J., and Fredrick, R.A., 2023, Response in the water quality of Delavan Lake, Wisconsin, to changes in phosphorus loading—Setting new goals for loading from its drainage basin: U.S. Geological Survey Scientific Investigations Report 2023–5073, 28 p., https://doi.org/10.3133/sir20235073.
ISSN: 2328-0328 (online)
Study Area
Publication type | Report |
---|---|
Publication Subtype | USGS Numbered Series |
Title | Response in the water quality of Delavan Lake, Wisconsin, to changes in phosphorus loading—Setting new goals for loading from its drainage basin |
Series title | Scientific Investigations Report |
Series number | 2023-5073 |
DOI | 10.3133/sir20235073 |
Year Published | 2023 |
Language | English |
Publisher | U.S. Geological Survey |
Publisher location | Reston, VA |
Contributing office(s) | Upper Midwest Water Science Center |
Description | Report: viii, 28 p.; Data Release; Dataset |
Country | United States |
State | Wisconsin |
Other Geospatial | Delavan Lake |
Online Only (Y/N) | Y |