Estimated Reductions in Phosphorus Loads From Removal of Leaf Litter in the Lake Champlain Drainage Area, Vermont
Links
- Document: Report (9.40 MB pdf) , HTML , XML
- Data Release: USGS data release - Data supporting phosphorus load-reduction estimates from leaf-litter removal in central and northwestern Vermont
- Download citation as: RIS | Dublin Core
Acknowledgments
The authors are grateful for the efforts of the students of the Applied Environmental Assessment and Analysis, Service Learning Project (ENSC 202) course (2018) at the University of Vermont, Department of Environmental Studies, for their assistance developing load-reduction credits. Special thanks to the many members of the public works departments of the nine participating municipalities who contributed their time and expertise. The authors also extend their gratitude to many U.S. Geological Survey (USGS) colleagues, including Alexander Bissell, Charles Sandusky, Meghan Santos, and Phillip Woodford, for assisting with sample processing in the laboratory; subject-matter experts Leslie DeSimone and Kirk Smith for technical assistance and reviews; and Judy Horwatich and Laura Medalie for their thoughtful technical reviews. The authors wish to express a special appreciation to our colleague and friend, Jarlath Patrick Macbeth O’Neil-Dunne, who died before this report was published. Jarlath was the Director of the Spatial Analysis Laboratory at the University of Vermont and Marine Corps veteran. His expertise developing the Urban Tree Cover protocols was instrumental to the development of the tree cover analysis used to calculate phosphorus load-reduction estimates.
Abstract
Excess nutrient loading and other factors are driving eutrophication and other negative effects on water-quality conditions in Lake Champlain and other receiving waters in Vermont. Two common best management practices were evaluated to determine how these practices can be optimized by targeting maintenance and operation to align better with seasonally driven needs, specifically to help municipalities remove a greater proportion of seasonal leaves and organic debris, reduce nutrient loading, and achieve water-quality goals.
To characterize solid materials typically removed by the municipal BMPs of catch-basin (CB) cleaning and street cleaning (SC), subsamples of CB and SC materials were collected each month from nine participating municipalities in central and northwestern Vermont between September 2017 and November 2018. Monthly and seasonal composites of CB and SC samples were created from the subsamples of available materials from all municipalities. Samples were analyzed for concentrations of total organic carbon, total Kjeldahl nitrogen, and total phosphorus (P), and separated into three particle-size fractions. Distribution of particle-size fractions was similar between CB and SC as both practices generally collect the coarser fraction of solid materials (greater than 125 micrometers in diameter). In the fall, however, the range of the coarser fraction of materials increased. This is attributed to the ability of SC to collect leaves and other light organic materials that commonly pass through a CB system designed to trap heavier materials.
Total organic carbon, total Kjeldahl nitrogen, and total P concentrations were highest in the catch-basin samples in the fall of 2017, and concentrations in the SC samples were highest in the fall of 2018. The collection of fewer samples in 2017 may account for some of the variability between fall 2017 and fall 2018 results. A subset of SC samples collected from piles representing specific street-cleaning routes in September and November 2018 were also analyzed. Materials collected in November were dominated by leaves, and the concentrations of the analyzed species of carbon, nitrogen, and phosphorus in some samples were more than double those in samples collected on the same street-cleaning routes in September.
The Vermont Department of Environmental Conservation and the University of Vermont developed estimates of load-reduction credits for CB and SC practices based on a policy developed by the Wisconsin Department of Natural Resources that determined the potential for credits associated with leaf-removal activities. This process also considered BMPs that were initiated during the U.S. Environmental Protection Agency’s Lake Champlain Basin Total Maximum Daily Load monitoring period (2000 to 2009) and adapted the Wisconsin Department of Natural Resources policies to apply to existing SC routes in the cooperating Vermont municipalities that possessed at least 17 percent tree cover. This exercise demonstrated that applying the Wisconsin Department of Natural Resources policy to existing street-cleaning routes possessing 17 percent or more tree cover would result in reductions in total P loads up to 65 percent of mandated target reductions, and about a 25 percent reduction on average.
Continuous simulations of stormwater runoff volume, and of loads of suspended sediments and total P, also were created for Englesby Brook Basin, an urbanized basin in Burlington and South Burlington that drains to Lake Champlain. Although the basin is more developed than the average of the nine cooperating municipalities, streamflow and P loading data collected by the U.S. Geological Survey were available to evaluate model performance. Simulations based on a year of average climatic conditions projected potential small reductions in total P of 0.08 to 0.10 percent as a result of CB cleaning and SC practices. Simulated weekly SC practices, however, reduced street-solid loads by as much as 7 percent. When the proportion of total P seen in fall SC materials collected in Vermont was applied to these simulated street-solid loads, estimated reductions of total P were about 29 percent. The combination of analytical results, estimated load-reduction credits, and simulated reductions indicate that targeted increases of SC activities to reduce leaf loading in the fall have the potential to reduce loading to receiving waters and could help regulated communities meet their water-quality goals.
Introduction
During periods of runoff of rainfall and snow meltwater, nutrients, other chemical constituents, and sediment wash off impervious surfaces and other source areas and ultimately drain to receiving waters such as rivers, ponds, and lakes. Excess nutrients facilitate increased algal and plant growth and lead to degradation of water quality and eutrophication. Excess sediment in stormwater runoff and meltwater is associated with large increases in concentrations of phosphorus (P) in the water, particularly in winter as a consequence of road maintenance activities (Smith and Granato, 2010).
Federally required limits on concentrations and loads of nutrients and sediment in runoff in the form of total maximum daily loads (TMDLs, https://www.epa.gov/tmdl) and municipal separate storm sewer systems (MS4s, https://www.epa.gov/npdes/stormwater-discharges-municipal-sources) are attempts to reduce nutrient and sediment loading, minimize eutrophication, and protect and improve water quality. P reductions that regulated communities must attain under the MS4 program can be greater than 60 percent and may include a requirement of meeting up to 20 percent of the P-reduction targets that must be met within the first 8 years of a permit term. Attaining these goals can require the reduction of tens to hundreds of kilograms of a constituent each year, and the costs associated with these reductions are high. To put this challenge in context, an evaluation of structural stormwater management options for communities in the upper Charles River Basin in Massachusetts reported an average estimated unit cost of about $85,000 per kilogram of P removed in the first 5 years (Horsley Whitten Group, 2011). Faced with this challenge, environmental managers need to consider all available options to reduce nutrient and sediment concentrations and loads to meet mandated goals. In urban or suburban settings with large areas of impervious surfaces combined with a large number of deciduous trees, the leaf-fall period provides a window of opportunity to reduce nutrient, carbon, and sediment loads to surface water. It has been well established that leaf matter and other organic detritus (grasses, flowers, pollen, and woody debris) contain high concentrations of carbon, nitrogen (N), and P and that the leaching rates of these constituents vary widely depending on tree species and climatic conditions (Cowen, 1973; Dorney, 1986; Waschbusch and others, 1999; Smith and Granato, 2010; Hobbie and others, 2013). Carbon plays an important role in the breakdown of organic matter and has climate resilience implications (Cai and others, 2010; Martialay, 2015). Removal of leaves and organic debris before exposure to rainfall can substantially reduce the dissolved fraction of carbon and nutrient loads, which commonly is not captured by most types of best management practices (BMPs). Recent work demonstrates that good housekeeping to prevent these types of organic materials from entering the stormwater drainage system, or the timely removal of materials that do enter the drainage system, is critical to minimizing nutrient loading into receiving waters (Smith, 2002, 2010; Hobbie and others, 2013, 2017; Selbig, 2016; Selbig and others, 2020).
Municipal stormwater control measures (SCMs) and BMPs are designed to maintain the cleanliness and aesthetic value of cities and keep their storm drainage infrastructure operating efficiently. Two common types of BMPs used in urban areas equipped with curbs and gutters are catch-basin (CB) cleaning and street cleaning (SC). Catch basins are structural BMPs whose design and installation can vary widely. For example, some catch-basin sumps are open and directly connected to the underlying soils to promote infiltration, and some are equipped with a hood over the outlet pipe to improve capture of floatable debris. Lack of maintenance of catch basins can contribute to reduced efficiency in achieving intended constituent-reduction goals (Smith, 2002, 2010).
Technologies for street cleaning, a nonstructural BMP, have advanced in recent decades. The traditional brush sweeper remains an essential tool for cleanup of large solids and organic material (such as leaves) from paved surfaces. Vacuum and regenerative-air technologies, which are used by some of the Vermont municipalities in this study, are more effective than brush sweepers at capturing the fine fraction of street solids and are capable of minimizing particulate matter entrained or ejected into the air. Voluntary or curbside leaf-litter removal programs are other nonstructural approaches that can reduce excess nutrient loading to receiving waters from developed catchments. As municipalities face the challenge of meeting federally mandated constituent-load reductions in the form of TMDLs and MS4s, a crediting system was developed by the U.S. Environmental Protection Agency (EPA) (Tetra Tech, 2016) to financially incentivize municipalities to implement load-reduction practices. Current (2020) credits allowed by the EPA Region I for CB, enhanced SC, and organic waste/leaf-litter collection programs are up to 2 percent, 10 percent, and 5 percent, respectively, of the total required load reduction (Tetra Tech, 2015, 2016; EPA, 2016). These credits, however, may not represent the full constituent-reduction potential of some of these common BMPs. Leaf litter is a source of nutrients long after leaves initially appear on street surfaces (Selbig and others, 2020), and recent studies have shown that targeted SC during leaf-fall periods can reduce annual nutrient concentrations by as much as 80 percent (excluding winter) (Selbig, 2016). By employing a leaf-litter collection program, annual nutrient loading may be further reduced (Hobbie and others, 2013; Kalinosky and others, 2014; Selbig, 2016; Janke and others, 2017). Selbig and others (2020), however, reported that leaf-collection programs alone showed no significant reductions in loads of total or dissolved P; remaining leaf materials on impervious surfaces contributed to an 83 percent increase in total N loads. The combined practices of leaf-collection programs and weekly SC in areas with high street-tree density were more effective at reducing nutrient loading than were only leaf-collection programs or structural BMPs (Selbig and others, 2020).
On the basis of responses to a national survey, Lager and others (1977) reported a decrease in the frequency of CB cleaning from twice per year in 1956 to once per year in 1973. Material entrained in CB sumps should generally to limited to 40 to 50 percent of sump capacity (Lager and others, 1977). Lager and others (1977) conducted simulations that showed accumulated sump materials did not reduce removal efficiencies until storage was 40 to 50 percent of sump capacity. Smith (2002), however, showed that reentrainment of sump materials occurred in a hooded deep sump at only 25 percent capacity during high-intensity rainfall events, and this reentrained material is available for loading to surface water.
Reported suspended-sediment removal efficiencies for CB with 4-foot (ft) sumps ranged from 35 to 90 percent over a flow range of 0.25 to 6.3 cubic feet per second (Lager and others, 1977). Aronson and others (1983) evaluated urban CB with sumps ranging in depth from 0.5 to 5 ft and reported suspended-sediment removal efficiencies ranging from −10 to +97 percent. Smith (2002) reported a suspended-sediment removal efficiency of 39 percent for deep-sumped, hooded CBs off a major urban highway in Boston, Massachusetts. Although there is always a need to maintain (or clean out) CBs, available municipal resources often make maintenance a low priority or wait until a blockage occurs (Lager and others, 1977). Additionally, current CB load-reduction credits of 2 percent in EPA Region I make it difficult for municipalities to justify redirection of limited resources to increase CB cleaning frequency.
The U.S. Geological Survey (USGS), in cooperation with the Chittenden County Regional Planning Committee, the Vermont Department of Environmental Conservation (VTDEC), the City of South Burlington, eight other municipalities in Vermont, and the University of Vermont, conducted an investigation of the solid materials collected by CB cleaning and SC BMPs to estimate the potential for reductions in nutrient loads by targeted applications of these BMPs. The Source Loading and Management Model for Windows (WinSLAMM, 1996 to 2018; Pitt and Voorhees, 2000; Pitt, 2008) was also applied to a small urban watershed in the greater Burlington area with available data previously collected by the USGS. Model scenarios were designed to simulate potential reductions of P and sediment loads that could be achieved with increased street-cleaning during leaf-fall periods as established by the State of Wisconsin (Wisconsin Department of Natural Resources [WDNR], 2022). The findings of that investigation are intended to facilitate the development of load-reduction credits that municipalities in central and northwestern Vermont can use to meet nutrient load-reduction goals and thereby improve receiving water quality.
Purpose and Scope
This report presents the physiochemical characteristics of solid samples collected from SC hopper materials, CB cleanout materials, and leaf litter during the fall of 2017 and during the spring, summer, and fall of 2018 from nine municipalities in central and northwestern Vermont (tables 1 and 2; fig. 1). A discussion of the sampling approach and of the results of the analyses of the samples, including particle-size fraction and quality-control samples, is also presented.
Table 1.
Acreage of land-use types and percent of total municipal area within the nine participating communities in the central and northwestern Vermont study area, and acreage of land-use types within the Englesby Brook Basin and percent of total basin area.[Based on NOAA 2006 C–CAP Regional Land Cover (Office for Coastal Management, 2020)]
Table 2.
Techniques used to manage and maintain catch basins and leaf litter in nine municipalities in central and northwestern Vermont.[U.S. Geological Survey (USGS) station identification numbers refer to locations where each municipality deposits catch-basin and street-cleaning materials that were subsampled each month during the 2017–18 study period. The Cities of Barre and Montpelier are not subject to municipal separate storm sewer system mandates. CB, catch basin; GIS, Geographic Information System; ANR, Agency of Natural Resources; lidar, light detection and ranging; --, no data]
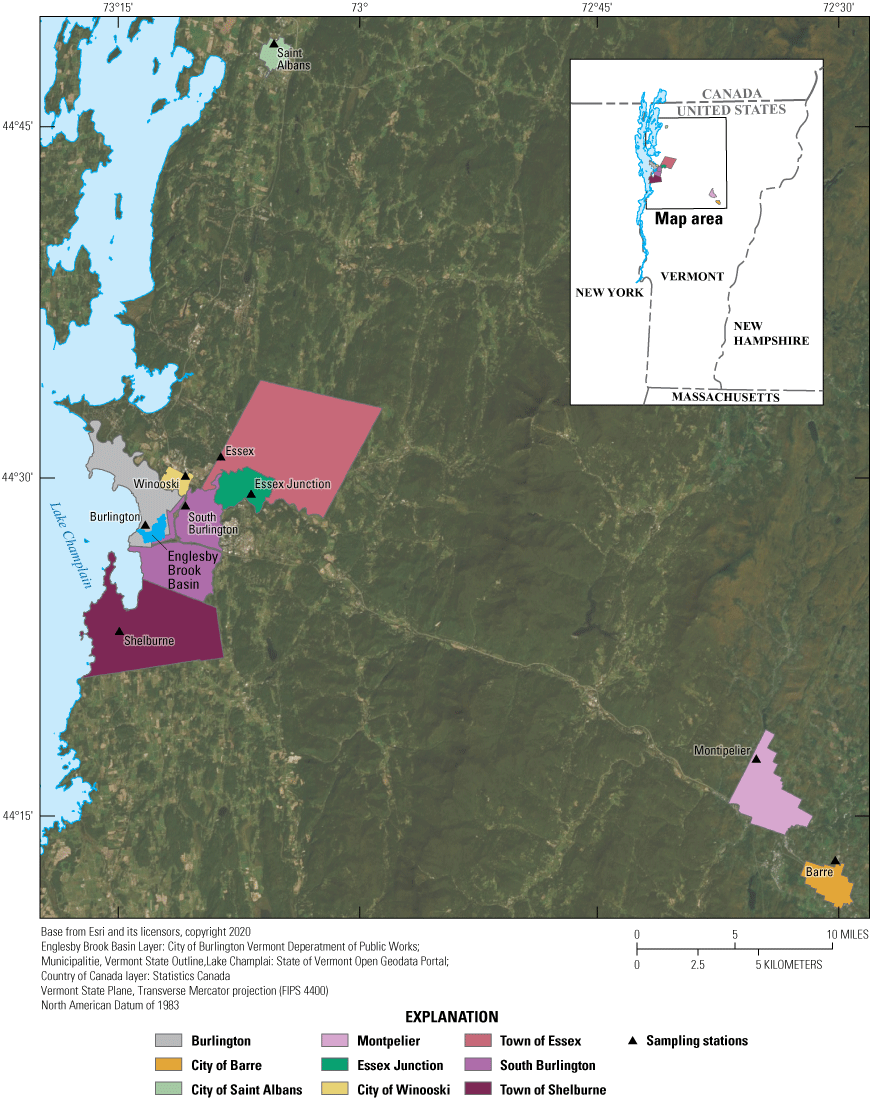
Map showing locations of the participating municipalities in central and northwestern Vermont.
The type and frequency of BMPs used by each municipality and their operation and maintenance practices are described. The potential for the reduction in nutrient loads that could be achieved by increasing the frequency of SC to better manage leaf loads and crediting policies based on a load-reduction crediting approach developed in Wisconsin are also discussed.
This report describes application of the Source Loading and Management Model for Windows (WinSLAMM) using land-use and other geospatial information and previously collected flow, precipitation, and water-quality data for a small urban watershed in northwestern Vermont. Alternative model scenarios that demonstrate potential reduction of P and sediment loads that could be achieved with increased SC frequency, as well as uncertainties associated with this model and analysis, are also discussed. Data used in support of the investigation are available to the public through the National Water Information System (U.S. Geological Survey, 2018) and in Sorenson and others (2024). Input and output files associated with the WinSLAMM application are provided in Sorenson and others (2024).
Effects of Leaf Litter Management on Phosphorus Loads
Algal growth in freshwater lakes is limited primarily by availability of phosphorus (P) (Wetzel, 2001). Much work has been done in recent years to reduce phosphorus loads from point sources such as wastewater treatment facilities and combined sewer overflows. Management of diffuse or nonpoint sources, however, will also help regulated communities meet mandated phosphorus reductions, control algal blooms, and improve overall water quality. Leaves and plant debris can be a large source of nutrients, carbon, and sediment in settings characterized by large areas of impervious surfaces. Cowen (1973) conducted P-leaching experiments using oak and poplar leaves soaking in distilled water. He reported that cut-up leaves released nearly three times as much soluble P as intact leaves and emphasized the importance of leaf-litter maintenance to reduce nutrient loadings to lakes. Work by Dorney (1986) investigated the leaching behavior of P from leaves of 13 different tree species in Wisconsin. Dorney (1986) reported that leachable P in leaves generally decreased with size and age of the tree. This phenomenon may be linked to fertilization at the time of planting or to older trees being more effective at translocation of P from the leaves before leaf fall. Concentrations of leachable P from air-dried, intact leaves, after soaking in distilled water for 2 hours to simulate urban runoff contact time, ranged from about 20 to 411 micrograms per gram (µg/g) air-dry weight, and the average concentration was 148 µg/g (Dorney, 1986). These results demonstrate that leaves can be a substantial source of P, particularly in urban centers characterized by substantial deciduous vegetation and large impervious areas, where leaves may remain on surfaces for days or weeks and be exposed to multiple rain events for periods much greater than 2 hours. Dorney’s work also showed the importance of knowing the concentrations of P in various tree species. He recommended the collection of leaves in urban areas with the highest P concentrations first, such as those dominated by Acer and Fraxinus (Maple and Ash) tree species versus areas dominated by Quercus and Tilia (Oak and Linden species) (Dorney, 1986). Understanding nutrient loading from specific tree species provides urban planners important information for targeting BMPs and urban plantings. Leaf-collection may be a useful nutrient-reduction approach in nondeciduous areas as well. Studies in Australia that examined leachable P and dissolved organic carbon from four tree species in an urban area showed that nonnative deciduous species drop most of their leaves within a short period in the fall, whereas native tree species exhibit constant leaf fall throughout the year (Wallace and others, 2008). Litter screens installed at the end of pipes or streams draining into receiving waters were effective traps for gross solids but were unable to prevent dissolved constituents like P and dissolved organic carbon from passing through or leaching from trapped materials within the first 48 to 72 hours of exposure to stormwater runoff (Wallace and others, 2008).
Breakdown of leaf litter from five tree species in an urban setting in Minnesota showed a nearly 80 percent loss of mass (ash free dry mass) within the first year (Hobbie and others, 2013). The experiments focused on decomposition rates in an urban gutter system, which were found to be much greater (only 20 to 40 percent initial mass remaining) than decomposition in nearby natural areas (50–85 percent initial mass remaining). Authors determined that 27 to 64 percent of all litter types consists of soluble materials. Leaves from all five species showed an initial rapid decomposition up to about 22 percent of the total mass, and all species (except Quercus bicolor, or swamp white oak) lost about 78 percent on average of their initial ash free dry weight after 1 year of decomposition. Most species retained more than half of their initial N mass, and exhibited N immobilization through microbial processes after 1 year (except oak). Losses in mass of P were characterized by a wide range in initial losses followed by several periods of P immobilization, then further loss at the end of the year. Swamp white oak and Norway maple species (Acer plantanoides) continued to immobilize P, while the three remaining species lost about 50 percent of their initial P mass loss at the end of the year. A double exponential model was applied to the mass-loss dynamics of an urban gutter system to describe the rapid initial loss of mass, small loss during winter (when snow-covered), followed by rapid loss after snow melt. These authors and others have demonstrated that nutrient exports vary in direct proportion to tree canopy cover (Selbig, 2016; Hobbie and others, 2017; Janke and others, 2017; Selbig and others, 2020).
Percent reductions in nutrient loads are also positively correlated with street-tree canopy (Selbig and others, 2020). A paired-basin study in Wisconsin demonstrated that 56 percent of the annual total P load was attributed to leaf litter in the fall (the study period did not include winter) and that intensive street cleaning during the leaf-fall period reduced total P and total N loading by as much as 84 and 73 percent, respectively (Selbig, 2016). This work helped the State of Wisconsin Department of Natural Resources (WDNR) develop a municipal phosphorus reduction credit for leaf management programs (WDNR, 2022) and support development of similar programs in other areas characterized by periods of annual deciduous leaf fall in autumn. In northern areas of the United States with substantial deciduous tree covers, large street-solid yields (which are often dominated by leaf litter) are seen in the fall, and the largest annual street-solid yields are seen at the end of winter, the period characterized by below-freezing temperatures when street cleaning has not occurred for several months (Selbig and Bannerman, 2007; Smith and Granato, 2010; Sorenson, 2013). Removal of this large mass of accumulated material could substantially reduce annual total P loading. Specifically, Selbig and others (2020) reported that weekly SC during the fall combined with leaf collection was the most effective approach to reducing annual P loadings.
A number of permitting programs and nutrient loading studies focused on Vermont and the Lake Champlain region are relevant to this study. Tetra Tech (2015) developed a detailed Soil Water Assessment Tool (SWAT) model to refine existing phosphorus TMDLs for the Lake Champlain Basin (EPA, 2016), to estimate achievable phosphorus load reductions and phosphorus loads from unmonitored areas, and to project phosphorus load changes due to changes to climate (EPA, 2016). The MS4 permit for the greater Lake Champlain area (nine municipalities, the University of Vermont, and Burlington International Airport), which was issued in 2018, requires the regulated communities to develop phosphorus control plans, including managing P from roadways, by 2021.
A crediting system to incentivize regulated communities currently (2020) used by the VTDEC is based on phosphorus reduction credits established in Massachusetts. If implemented fully, load-reduction credits of between 2 to 10 percent are available for street cleaning and 2 percent for catch-basin cleaning (Massachusetts Department of Environmental Protection, 2016). In the greater Lake Champlain area, some street cleaning and other BMPs practices were already in place prior to establishment of the TMDL and MS4 permits in 2010. Therefore, VTDEC has awarded prorated credits of 10 percent per year for practices that started between 2000 and 2009 (referred to as the TMDL monitoring period).
Several studies demonstrate the potential effectiveness of structural and nonstructural BMPs in the Lake Champlain Basin in Vermont. The Englesby Brook Restoration Plan (Stone Environmental, 2017), an assessment of existing and proposed BMPs, included a flow restoration plan (FRP) and a planning tool for an impaired urban catchment. A similar FRP project was conducted for Potash Brook in South Burlington (City of South Burlington, 2016). This FRP examined the use of structural BMPs such as gravel wetlands and infiltration basins and was updated in 2019. The U.S. Geological Survey (USGS) investigated the effectiveness of BMPs in urban and rural Lake Champlain tributaries on reducing concentrations and loads of nutrients and suspended sediment (Medalie, 2007 and 2012). The Vermont Department of Transportation published a study of structural BMPs to help identify criteria and stormwater management options to address postconstruction runoff (Vermont Department of Transportation, 2012).
Study Area
Solid materials, including leaves and other organic detritus, were collected from nine municipalities (communities) within central and northwestern Vermont (fig. 1). Seven of these communities are completely within the Lake Champlain drainage basin; these seven communities include some of the most populous cities in Vermont: Burlington, South Burlington, and Essex Junction. These seven communities are required to abide by TMDL and MS4 regulations to minimize nutrient loading to Lake Champlain (EPA, 2016). The remaining two communities, Montpelier and Barre, are not subject to TMDL and MS4 regulation but employ similar BMP practices. A summary of the distribution of land-use types within all nine communities is presented in table 1.
Tetra Tech (2015) prepared a model for the Lake Champlain Basin to develop phosphorus loading estimates by using the SWAT and applied hydrologic soil groups developed by the U.S. Department of Agriculture, Natural Resources Conservation Service (2009). The hydrologic soil groups were used to categorize infiltration capacity in the Lake Champlain Basin from highest (A, resulting in the least potential runoff) to the lowest (D, resulting in the most potential runoff). Tetra Tech (2015) reported percentages of land area in the Lake Champlain Basin falling into the A, B, C and D soil groups are 11.0, 19.9, 34.5 and 34.6 percent, respectively. The surficial geology of the area consists primarily of deposits of silt and clay from marine and lake settings (Wright, 2003), which explains the relatively large proportion of the total area falling into the soil groups with lower infiltration capacity. National Weather Service, National Climatic Data Center records from the Burlington International Airport in South Burlington show the average annual precipitation for 128 years of record (through 2013) is about 91 centimeters (cm) and for the last 13 years of that record is about 98 cm (National Weather Service, National Climatic Data Center, 2019). Climatic and soil-infiltration capacity data were used to guide the development of the WinSLAMM model.
Collection and Analysis of Samples
The type and frequency of catch-basin cleaning and street cleaning employed by each of the nine Vermont municipalities and other ancillary information are listed in tables 2 and 3. These tables do not include information about other structural BMPs that may have been installed in each community. The reported catch-basin cleaning frequencies ranged from 1 to 9 years (table 2). Street-cleaning frequencies ranged from weekly, for high-traffic/high-pedestrian use streets such as those in downtown areas, to annually (table 3). Many of the municipalities have recently purchased vacuum or regenerative-air types of street cleaners as well. Most of these nine communities have an area in their public yards or transfer stations available for residents to drop off bagged leaves. The cities of Burlington and Barre operate curbside leaf-collection programs, but it is unclear what proportion of their residents participate in those programs. USGS sampling stations were established in each community and are also listed in table 2 and shown in figure 1.
Table 3.
Frequency and type of street cleaning used in nine municipalities in central and northwestern Vermont.[U.S. Geological Survey (USGS) station identification numbers refer to locations where each municipality deposits catch-basin and street-cleaning materials that were subsampled each month during the 2017–18 study period. W, weekly; --, no data; Y, yearly; 2X, twice per year; M, monthly; 2x+, twice per year or more; --, no data]
Composite Samples
Solid materials that were collected from CB and SC by each participating municipality between September 2017 and November 2018 as part of their normal operations represented the raw materials subsampled for this study. Piles of CB and SC solids were deposited by public works operations in designated areas or at transfer stations within each municipality (fig. 1; table 2). These materials were not available for sampling during the months of December through March because these maintenance programs are suspended for the winter. When materials were available, subsamples from the deposited piles in each municipality were collected at the end of each month. A simple random sampling approach (EPA, 1994) was used to create composite samples, following methods similar to those described by Smith (2002) and Smith and Granato (2010). Initially, the proportion of organic debris in a pile of materials was estimated, and this estimate determined the number of subsamples to be collected from that pile. A maximum of 20 subsamples were collected if organic debris (defined as pollen, flowers, leaves, sticks, and other types of plant and woody debris) made up more than 80 percent of the pile for that month. As the proportion of organic debris decreased, the number of subsamples collected to create the composite samples was also decreased. For example, if 30 to 50 percent of the pile was composed of organic debris, then 10 subsamples were collected. If less than 10 percent of the pile was organic debris, then a minimum of 5 subsamples were collected, as the variability in the material would be considered much less compared to piles containing a large proportion of organic debris.
Steel shovels were used to collect the subsamples, which were placed in dedicated, labeled (for example, Essex Junction-August catch-basin or Montpelier-August street-cleaning materials), 5-gallon white plastic buckets with lids. Shovels were cleaned between each sampling site by following the same procedure as that for cleaning the sampling buckets: washing with a nonphosphate detergent solution followed by tap and deionized water (DIW) rinses. The first areas or test pits that were dug with the cleaned shovel at each new pile were not included in the subsample. The buckets and lids used for each month’s composite sampling, as well as plastic mixing trays, were precleaned in the laboratory with a nonphosphate detergent solution followed by tap water rinse, then allowed to soak in 5-percent hydrochloric acid solution (5-percent HCl) and DIW rinses until the specific conductance of the rinsate was equal to or less than the water coming directly from the DIW system (less than 1 microsiemens per centimeter at 25 degrees Celsius).
Composite subsamples of each monthly pile of municipal CB and SC materials were deposited into a 5-gallon bucket, sealed, and transported to a USGS New England Water Science Center laboratory. Subsamples were mixed thoroughly by hand using stainless steel tools until the mixture reached a consistent texture and color (Breault and others, 2013). Anthropogenic trash (such as glass, plastic, metals, foam, and cigarettes) was removed from the homogenized composites, and two composite subsamples were collected from the mixture for (1) particle-size analysis and (2) chemical analysis. The target analytes and techniques used for chemical analyses are shown in table 4. Samples to be analyzed for solid materials were shipped to the USGS National Water Quality Laboratory (NWQL) contract laboratory RTI in Livonia, Michigan. Samples were not pulverized at USGS request because of concern that extreme crushing process on the sample could add material from the matrix of rock, concrete, and asphalt to the samples and bias the results compared to what is typically available to leach off of materials on street surfaces and in catch-basin sumps without crushing. The contract laboratory’s analytical chamber is also small, however, and when samples contained large pieces of organic debris, the larger leaves and woody debris had to be cut into smaller pieces to ensure that the composited subsample would fit into the small analytical container used for total organic carbon (TOC) analysis (table 3). Torn up or crushed leaves leach nutrients more quickly than do whole leaves (Dorney 1986; Hobbie and others, 2013), and leaves on streets may be broken down by car tires and other physical actions before they reach receiving waters. The influence of pulverized gravel, asphalt, metals, and small, unseen bits of anthropogenic trash (plastic, glass, Styrofoam, and so on) on the chemistry of street runoff is unknown. With this in mind, samples that are only minimally pulverized may better represent concentrations of chemical constituents and organic compounds available from the solid materials that are washed off streets and into Lake Champlain and intermediate receiving waters.
Table 4.
Target analytes and techniques used to analyze subsamples of catch basin, street cleaning, and leaf litter solid materials collected in nine municipalities in central and northwestern Vermont; and water samples used to estimate concentrations in stormwater containing leaves and organic debris.[MDL, method detection limit; ICP–MS, inductively coupled plasma-mass spectrometry; SW, solid waste; EPA, U.S. Environmental Protection Agency; mg/kg, milligram per kilogram; SM, standard methods; ASTM, American Society for Testing and Materials; mg/L, milligram per liter; HTC, high-temperature combustion]
To minimize the inherent variability associated with the composition of the solid material samples, as well as the variability in that composition among the nine communities, monthly composites of CB and SC materials were aggregated by month before chemical analysis. Averages were then computed by using monthly sample results within three seasonal study periods: (1) spring, which includes only the month of May; (2) summer, which includes June, July, and August; and (3) fall, which includes September, October, and November. It is important to reiterate that most CB and SC operations were suspended between December and April because freezing temperatures impede these water-dependent activities. CB cleanout materials were less abundant than SC materials during the study period.
Leaf samples collected from bagged leaves brought to the South Burlington transfer station by residents were submitted to RTI labs for the solid materials analyses indicated in table 4. These leaf samples also were used in laboratory experiments at the USGS New England Water Science Center laboratory in Northborough, Massachusetts, to estimate nutrient concentrations that might be expected in rainwater runoff exposed to leaves and pine needles. Leaves from various species of deciduous trees and pine needles were collected by field personnel. Three known masses of deciduous leaves were placed into precleaned 4-liter (L) high-density polyethylene plastic containers filled with rainwater. Three known masses of pine needles also were placed into three similar 4-L plastic containers filled with rainwater, which was collected in precleaned high-density polyethylene bins overnight during the summer. The rainwater was decanted from each of these containers after the leaf and pine needle masses were allowed to soak for 1-hour, 2-hour, and 22-hour periods. These water samples, as well as a sample of raw rainwater, were shipped to the USGS NWQL in Denver, Colorado, for analysis of total and dissolved constituents (table 4).
Quality-Control Sampling and Processing
Field blank and field replicate samples were collected with regular CB and SC solid material (environmental) samples and processed following similar procedures to assure that manual and composite sampling equipment was not a source of contamination bias and that sampling and laboratory procedures resulted in reproducible samples of these solid materials. All blank and replicate samples were processed at the USGS New England Water Science Center laboratory in Northborough, Massachusetts, or in Montpelier, Vermont. Both the quality-control samples and environmental samples were shipped to the USGS contract lab RTI in Livonia, Michigan, for the analysis of the constituents (analytes) listed in table 4. Additional sample results of blank material from other USGS studies are also discussed.
Blank Samples
Samples of dry, graded, unground silica sand (sourced from Ottawa, Illinois [U.S. Silica]) were analyzed for total Kjeldahl nitrogen (TKN), total P, and TOC (table 5). Initial analyses of samples of the dry sand indicated concentrations of TOC and TKN that were considered too high and thus not appropriate to use as a blank material for these constituents, but total P values were low. To ensure the sand did not introduce TOC and TKN contamination and to determine if cleaning and processing protocols were sufficient to minimize contamination bias, two silica-sand samples were allowed to soak in 5-percent HCl for about 48 hours, then rinsed with DIW until the specific conductance of the rinsate was equal to or less than that of the water coming directly from the DIW system, as described above. One half of the HCl-rinsed blank material was set aside and the other half was dried at 60 degrees Celsius (°C) for 48 hours. Both wet and dry sand samples were exposed to a clean shovel, placed in a plastic 5-gallon buckets, and then stored in a dedicated plastic sampling bags and stored for 48 hours at 4 degrees °C in a similar manner as were the municipal-solid samples collected in the field. The sand samples were then dried at 60 °C to constant mass in precleaned stainless steel trays and emptied into precleaned stainless steel sieves and shaken for 30 minutes. Concentrations of TKN in the dried and wet HCl-rinsed equipment-blank samples were equal to or about 3.5 times greater than the minimum TKN concentration observed in environmental samples. TKN concentrations were also less than the 10th percentile of TKN concentration in all in CB or SC environmental samples. Total P concentrations were about three to four times less than the lowest concentration observed in SC or CB samples, and TOC concentrations in both of the blank samples were below the method detection limit. Results of the analyses of the two HCl-rinsed blank samples indicate there may be some positive bias in TKN concentrations but that sampling and processing equipment and sampling handling methods were not a significant source of contamination for total P and TOC.
Replicate Samples
Results of the analyses of samples of solids and sediment are known to be highly variable, and relative percent differences (RPDs) between concentrations of constituents in replicate samples of sediment are not unusual. Smith (2002) reported RPDs in concentrations of TOC in some replicate samples of sediment from highway catch basins exceeded 50 percent, and RPDs in concentrations of total P in solids collected from streets in Cambridge, Massachusetts, ranged from zero to 111 percent (Sorenson, 2013). Breault and others (2000) considered RPDs of 50 percent or less between constituent concentrations in replicate samples of aquatic sediment, which consist primarily of fine particles, to be acceptable.
Replicate samples of CB and SC materials were collected from the Vermont communities on two separate dates in the fall of 2017. Two subsamples (shovel loads) were collected from each subsample location within each pile of CB or SC material and distributed between two dedicated and precleaned composite sample buckets. Three pairs of split-replicate samples of SC material, one pair from each of three particle-size fractions (defined below), were collected from the fall 2018 composite SC sample. Results of the analyses of all replicate sample pairs collected in 2017 and 2018, as well as the RPDs between constituent concentrations in the samples for each pair, are shown in table 6.
Table 6.
Relative percent differences in constituent concentrations in replicate subsamples of catch-basin and street-cleaning materials collected by nine municipalities in central and northwestern Vermont.[Total sample results are reported in wet weight, and results for replicate pairs of the three particle-size fractions are reported in dry weight. mg/kg, milligram per kilogram; CB, catch basin; RPD, relative percent difference; SC, street cleaner; Coarse, materials greater than or equal to 2 millimeters (mm); Medium, less than 2 mm to greater than or equal to 0.125 mm; Fine, less than 0.125 mm in diameter; --, no data]
RPDs in concentrations of TOC in field replicate samples were 92.6 and 30.3 percent. And RPDs in TOC concentrations in split replicates were 92.1, 8.33, and 2.41 percent in the coarse, medium and fine fractions, respectively. RPDs in concentrations of TKN in total samples were 13.3 and 38.9 percent, and RPDs were 142, 20.0, and 4.65 percent in the coarse, medium, and fine particle-size fractions, respectively. RPDs in concentrations of total P in total and fractionated replicates were all less than 10 percent. These results indicate that field and laboratory methods produced the least variability in total P sample results. RPDs greater than 30 percent in TOC and TKN concentrations, however, were associated with sample pairs of total material collected in the field and from the coarse-fraction replicates that contained the largest proportions of highly variable organic materials, such as leaves and woody debris. For example, of the two RPDs in concentrations of TOC that were about 92 percent, one was from the coarse fraction sample pair, which was also associated with the highest RPD for TKN of about 142 percent (table 6).
Particle-Size Fraction and Moisture Content
Stainless-steel sieves and a mechanical sieve shaker were used to split subsamples of monthly CB and SC composite samples into three particle-size fractions: (1) coarse, greater than or equal to 2 millimeters (mm) in diameter; (2) medium, less than 2 mm and greater than or equal to 0.125 mm in diameter; and (3) fine, less than 0.125 mm in diameter. Initially, a wet-sieving process was attempted to help preserve nitrogen that could be subject to volatilization losses through oven drying, and to provide four particle-size fractions (less than 0.063 mm in diameter) rather than three size fractions resulting from mechanical sieve shaking (less than 0.125 mm in diameter). The wet-sieving approach was time consuming, leachate from sieve waters likely contained target analytes, and results of the finer fractions may have a high bias (Colman and Sanzolone, 1991). In addition, it was not possible to determine the proportion of leachate from specific particle-size fractions. For these reasons, subsamples of monthly solids were dried at 60 °C to constant mass, then split into three particle-size fractions by using a mechanical sieve shaker. The relative proportions (percentages) of the solid materials in each of the particle-size fractions of all subsamples taken from monthly composites of CB and SC materials collected by the study area municipalities are shown in table 7. For CB and SC samples, medium-sized particles are the most abundant and fine-sized particles are the least abundant by mass (fig. 2).
Table 7.
Distribution of total sample mass across three particle-size fractions in catch-basin and street-cleaning materials collected in nine municipalities in central and northwestern Vermont.[Monthly and seasonal average distributions from available materials are also shown. 2017 values are based on analysis of total samples and 2018 values are based on the sums of concentrations weighted by particle size fractions. Street-cleaning materials specific to designated maintenance routes from the fall of 2018 from two municipalities are also shown. Coarse, particles greater than or equal to 2 millimeters; Medium, particles less than 2 to greater than or equal to 0.125 millimeters; Fine, particles less than 0.125 millimeters in diameter; B, City of Barre; SB, City of South Burlington; --, not applicable, as rows for monthly and seasonal averages represent multiple stations and one or more sample dates]
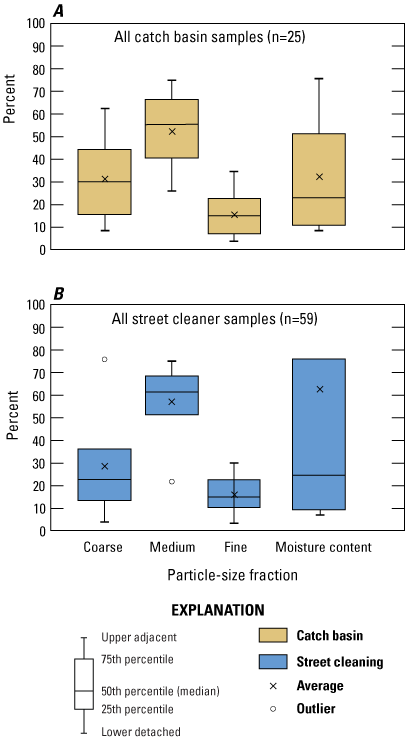
Graphs showing particle-size distribution and moisture content in samples of A, catch-basin and B, street-cleaning materials collected by participating municipalities in central and northwestern Vermont.
Smith (2002, 2010) demonstrated that catch basins and mechanical brush street-cleaning BMPs both target primarily medium to coarse materials. Smith (2002) also reported the primary benefit of mechanical street cleaning is the capture of solids greater than 8 mm in diameter. This larger fraction commonly includes organic debris and floatables that likely pass through a CB system. Yet the distribution across three particle-size fractions in samples of both CB and SC materials collected in the Vermont municipalities shows little difference between the two BMP types (fig. 2). The most apparent difference between the CB and SC samples is that moisture content is higher in some SC samples than in CB samples (fig. 2). Similar distributions are seen in seasonal results (fig. 3), but whereas particle-size distributions in CB samples become narrower in the fall, they become wider in SC materials. Street-cleaning technologies such as vacuum-assisted and regenerative air have the capability to capture finer fraction solids compared to mechanical brush SC technology, but this capability is commonly reduced by the need to apply water to minimize the generation of airborne particulates and resulting poor air quality (Selbig and Bannerman, 2007; Sorenson, 2013). General types of street cleaners used by the nine Vermont communities are shown in table 2.
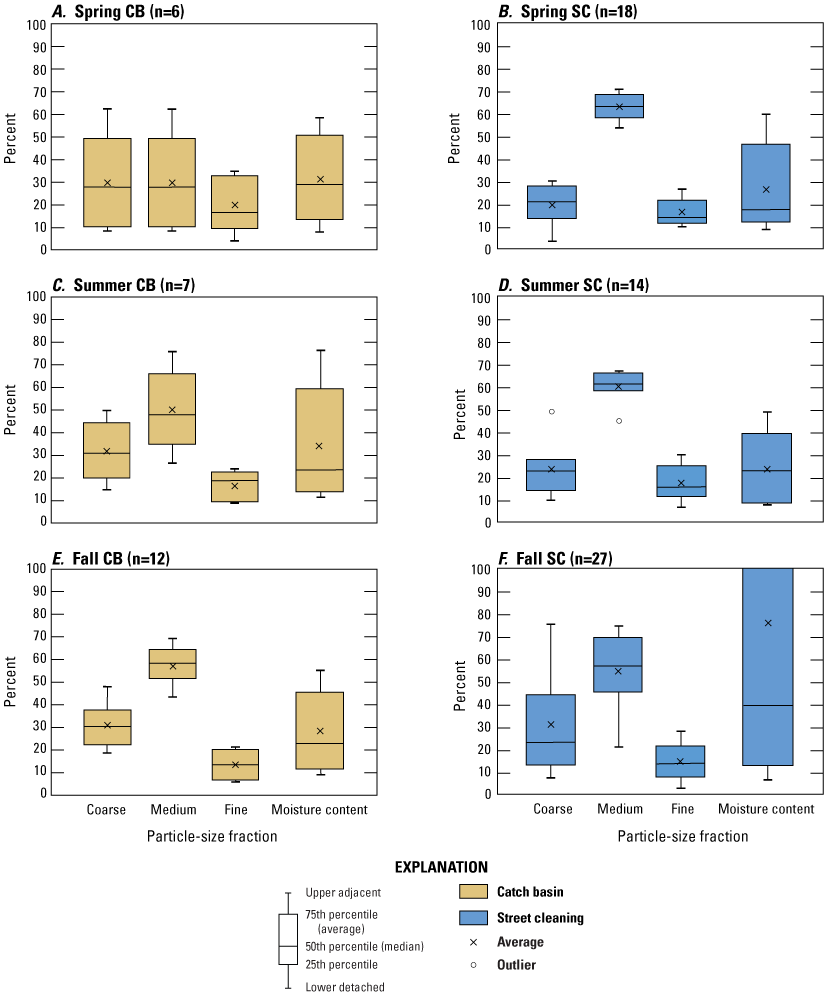
Graphs showing particle-size distribution and moisture content in seasonal composite samples of catch-basin (CB) and street-cleaning (SC) materials collected by participating municipalities in central and northwestern Vermont. A, Spring CB samples; B, spring SC samples; C, summer CB samples; D, summer SC samples; E, fall CB samples; and F, fall SC samples.
Sources of inherent bias in the materials sampled in Vermont include water content and loss of the fine fractions in CB materials during the vacuum water-excavation process used to clean CB sumps. CB cleanout materials, collected at various frequencies and including water added during sump maintenance to scour sediments, were brought back to town yards by municipalities or their contractors for temporary storage. Sediment-laden water was usually decanted on site before depositing CB solids in their designated areas. It is likely that some of the finer fractions of sediments were not included with the decanted sample and that the particle-size distributions are accordingly biased toward larger particles. In general, both CB and SC activities do not effectively collect or trap finer fractions of solid materials, and the higher volumes of organic debris in SC materials, particularly in the fall, may result in seasonally high concentrations of nutrients and carbon.
Both CB and SC materials were exposed to ambient conditions, which included wet and dry weather, for as long as a month before subsamples were collected. Many organic materials can retain water in pore spaces, which may explain the higher moisture content seen in SC materials compared to CB materials, particularly in the spring and fall (figs. 2 and 3), because organic debris and leaves typically float in water and pass through a CB rather than being captured in the sump.
Nutrient and Carbon Concentrations
Subsamples of CB and SC solids were collected each month from September 2017 through November of 2018, as described in the ”Composite Samples” section. From 5 to 25 subsamples from each available CB and SC pile in the 9 communities were weighted equally and composited each month to collectively represent the materials removed from catch basins and streets by these two BMP types. Thus the results of analyses of these samples for their carbon and nutrient content represent the concentrations of these constituents available for washoff from road surfaces (or contained in CB sumps) within the participating municipalities during that month. Monthly sampling events resulted in composites representing 24 individual SC samples and 15 CB samples (table 8). An additional 13 route-specific SC samples, as well as quality assurance and replicate samples, were also collected. Leaf-litter subsamples also were collected in October 2017 from a drop-off area in South Burlington where residents brought bagged leaves and yard waste materials. Results of the analyses of route-specific SC samples were used to evaluate the effects of tree cover on concentrations of carbon and nutrients.
Table 8.
Results of analyses of catch-basin and street-cleaning materials collected by nine municipalities in central and northwestern Vermont.[2017 values are based on analysis of total samples, and 2018 values are based on the sums of concentrations weighted by particle size fractions. Leaf-litter samples were collected from bagged leaves brought to a public yard by residents. Monthly composite samples were partitioned into three particle-size fractions. Values for total samples in 2017 represent analyses of wet, unseparated materials. Values for total samples and separate particle-size fractions in 2018 represent results of analyses of dry material. mg/kg, milligram per kilogram; Total, total monthly value; --, no data; Average, seasonal average value; Coarse, materials greater than or equal to 2 millimeters (mm); Medium, less than 2 mm to greater than or equal to 0.125 mm; Fine, less than 0.125 mm in diameter]
Results of the analyses of monthly composite samples of CB, SC, and leaf-litter materials are shown in table 8. Total, unseparated, and undried samples composited from all 9 communities for each of the three months in the fall of 2017 were submitted for chemical analysis and dried by the receiving lab. The averages for the dry-weight concentrations of TOC, TKN, and total P in these monthly samples are the seasonal concentrations for the fall of 2017. Subsamples from all available materials collected by each community each month were combined into a monthly composite sample for CB and SC in 2018. Monthly 2018 composites were dried then separated into three particle-size fractions before each fraction was submitted for individual analysis. Concentrations were weighted by each of the three size fractions and summed to provide monthly totals. The average of the monthly totals provided the seasonal concentrations for the spring, summer, and fall of 2018. The percent that each fraction contributed toward the total mass was also calculated, and estimates of the proportions of TOC, TKN and total P within each of the three particle-size fractions and the total of monthly CB and SC materials are also shown. Samples collected in 2017 were submitted without drying, and particle-size fractions separated by wet-sieving were not submitted to the laboratory for analysis due to concerns of high bias in the smaller fractions. Colman and Sanzolone (1991) discussed the inability to allocate wet-sieve water leachate concentrations to a particular size fraction before being submitted for laboratory analysis. In 2018, however, samples were dried and then mechanically separated before being submitted for analysis. The receiving laboratory dried the wet samples that were submitted in 2017, which allowed for comparison between 2017 and 2018 sample results in dry weight.
The concentrations of TOC, TKN, and total P in CB and SC materials on both a seasonal and monthly basis are shown in figure 4. Concentrations of these constituents are slightly less sensitive to seasonality in CB materials than in SC materials because CB sumps typically are cleaned only once per year or less and the analytical analyses reflect carbon and nutrient content of materials integrated across several seasons. The highest CB and SC concentrations for TOC, TKN, and total P are seen in the fall on both the seasonal and monthly plots, with the highest concentrations generally in the fall of 2017 (fig. 4). Both 2017 and 2018 sets of monthly SC concentration data also show similar patterns during the sampling period. The largest concentrations of all three analytes were in the two fall periods, and the lowest concentrations were in the summer.
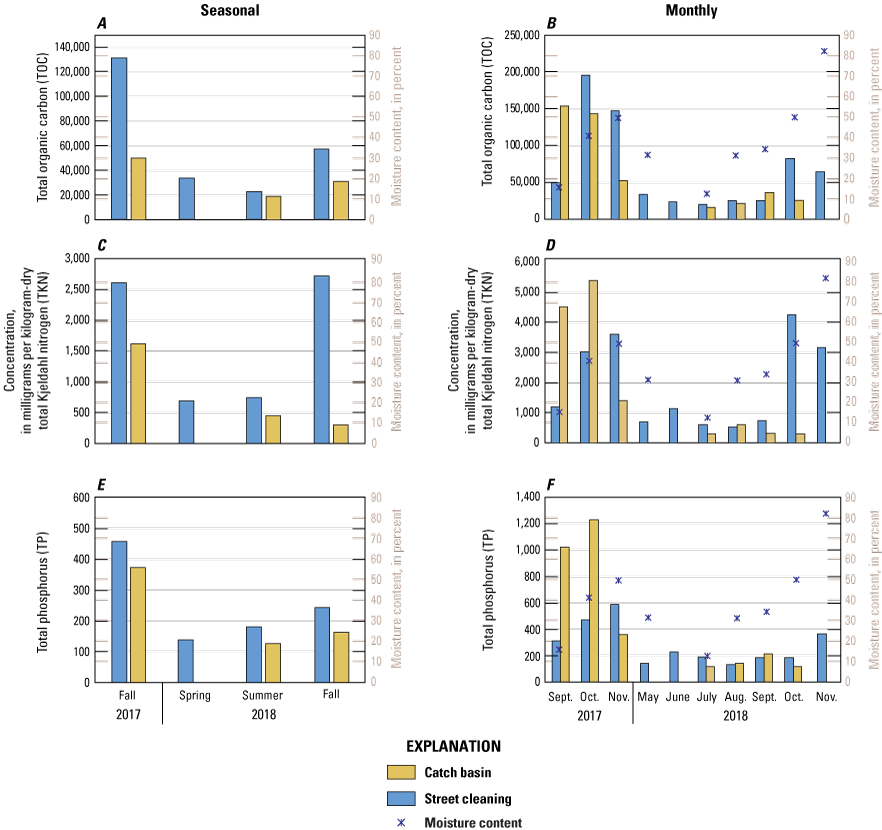
Graphs showing seasonal total composite and monthly total composite concentrations of carbon and nutrients in catch-basin and street-cleaning materials collected by participating municipalities in central and northwestern Vermont. A, seasonal and B, monthly total organic carbon (TOC) composite concentrations; C, seasonal and D, monthly total Kjeldahl nitrogen (TKN) composite concentrations; and E, seasonal and F, monthly total phosphorus (TP) composite concentrations. Note that 2017 values are based on analysis of total samples, and 2018 total values are based on the sums of concentrations weighted by grain-size fraction.
The proportions of the total mass and concentration ranges of all three analytes are greater in SC materials than in CB materials but are greatest for TKN for both total material and in all three particle-size fractions (fig. 5). The next greatest difference in proportion of analytes between SC and CB material is in the coarse fraction of total P, followed by coarse and medium fractions of TOC. It is likely that the coarsest fraction of collected material (particles greater than 2 mm diameter) is greater in SC materials than in CB materials because SC material has more large-diameter organic debris, such as leaves, compared to CB materials. To develop estimates of stormwater constituent loads associated with structural BMPs, CB and SC materials were sampled by the City of South Burlington for particle-size analysis and for analysis of concentrations of total N, total P, and total suspended solids (Fitzgerald Environmental Associates LLC, 2018). Although the South Burlington study differed in details from this study and was smaller in scope, in 1 to 4 samples from CB- and SC-material piles, and in sample materials that were milled to a consistent size fraction, the resulting average CB and SC total P concentrations (283 and 390 mg/kg, respectively) and average proportion of total P in CB and SC materials (0.028 and 0.039 percent, respectively) were similar to those seen in this study (table 7) (Fitzgerald Environmental Associates LLC, 2018).
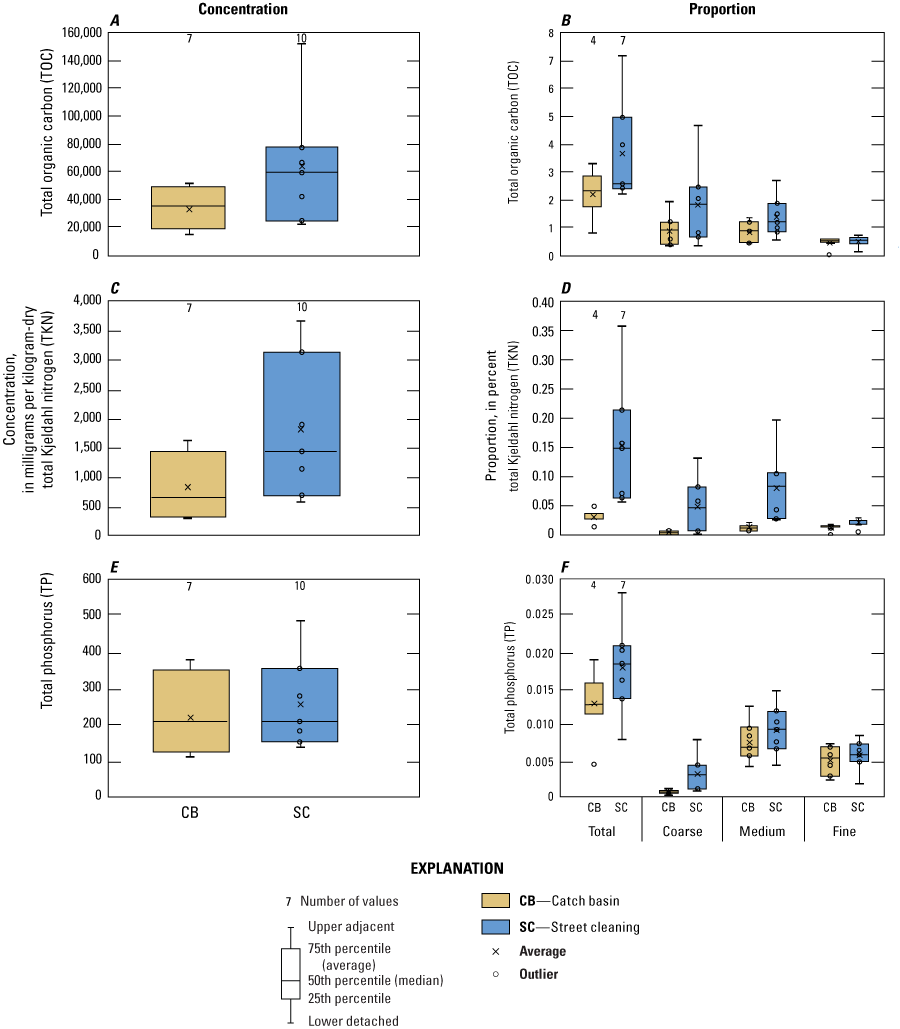
Graphs showing distribution of total composite concentrations of carbon and nutrients in catch-basin (CB) and street-cleaning (SC) materials and estimated proportions of total organic carbon and nutrients in the total mass and within three particle-size fractions in materials collected by participating municipalities in central and northwestern Vermont. A, total organic carbon (TOC) composite concentrations; B, estimated proportions of TOC; C, total Kjeldahl nitrogen (TKN) composite concentrations; D, estimated proportions of TKN; E, total phosphorus (TP) composite concentrations; and F, estimated proportions of TP.
In the fall of 2018, the city of Barre and the city of South Burlington deposited SC materials in their city yards in route-specific piles for subsampling. Results of analyses of samples collected from these route-specific composites in September and November 2018 are presented in table 9 and graphically in figure 6. Materials were only available in both cities in September, and concentrations for all three analytes (TOC, TKN, and total P) were comparable between the two municipalities. Analysis of samples collected from Barre in November indicated a significant increase in concentrations of all three analytes compared to those collected in September (table 9 and fig. 6). Visual inspections of piles of street-cleaning material collected from Barre in September and November show a large increase in leaf matter and other organic debris in November (two examples of piles are seen in figure 7). Percent tree cover in the street-cleaning Route 3 area is one of the highest in South Burlington’s street-cleaning route groups, and samples collected in September contained some of the highest concentrations of all three analytes. Tree cover was greater than 17 percent in most of Barre’s street-cleaning routes (table 9), and of the three routes sampled in September and November, the samples collected in November show a 2- to nearly 30-fold increase in total P (table 9). Results for these samples agree with the established correlation between the percentage of tree cover and nutrient loading in stormwater runoff (Waschbusch and others, 1999; Hobbie and others, 2013; Baker and others, 2014; Selbig, 2016; Janke and others, 2017; Selbig and others, 2020) and further support the potential for street-cleaning and leaf-litter removal activities as a means to help MS4 communities to meet nutrient load-reduction targets.
Table 9.
Results of analyses of samples of street-cleaning materials collected along specific routes in the City of South Burlington in September 2018 and the City of Barre in September and November 2018.[SWAT, Soil Water Assessment Tool; mg/kg; milligram per kilogram; ID, identifier; <, less than; --, no data]
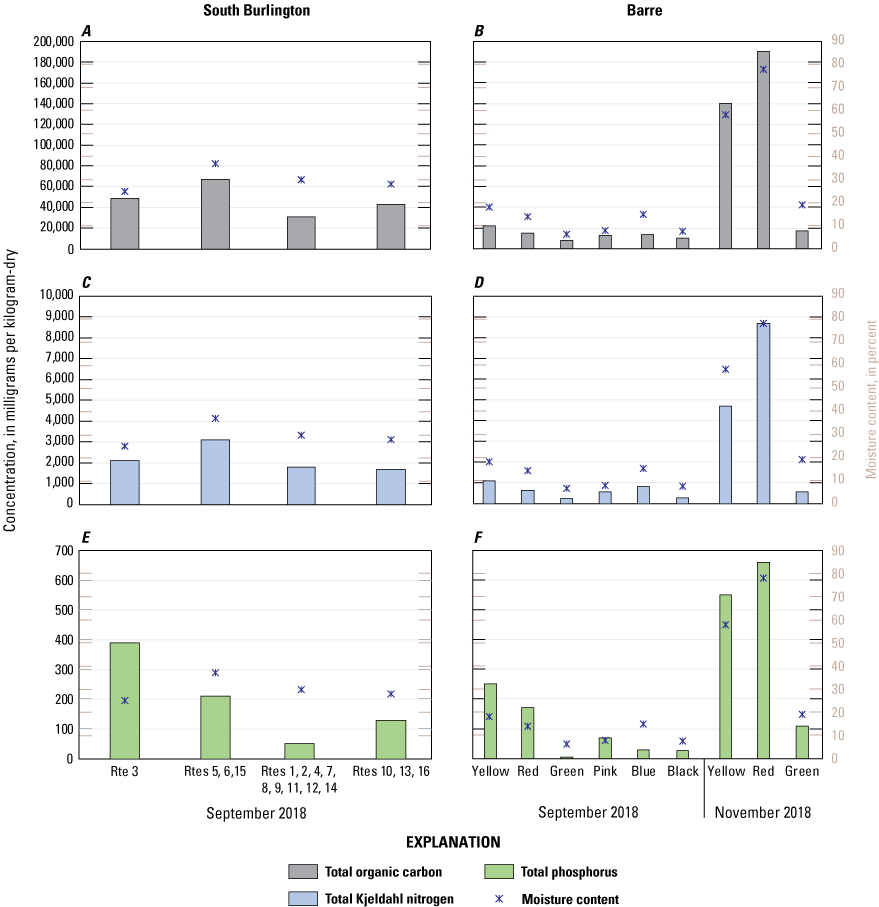
Graphs showing carbon and nutrients in street-cleaning materials from route (Rte)-specific streets in the city of South Burlington in September 2018 and the city of Barre in September and November 2018. Total organic carbon in A, South Burlington and B, Barre; total Kjeldahl nitrogen in C, South Burlington and D, Barre; and total phosphorus in E, South Burlington and F, Barre.
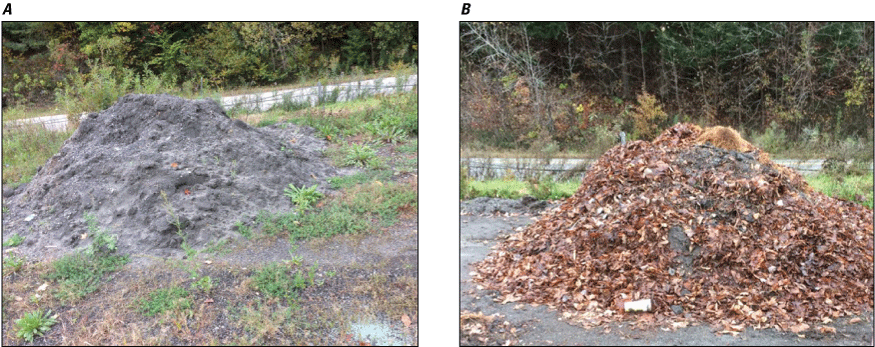
Photographs showing examples of street-cleaning materials collected along specific routes in the city of Barre in A, September and B, November 2018.
A sample of rainwater and five samples of rainwater in which known masses of pine needles and known masses of deciduous leaves were allowed to soak for 1-hour, 2-hour, and 22-hour periods (only deciduous leaves were soaked for 22 hours) were analyzed for their carbon and nutrient content. The results of these reconnaissance-level analyses provide estimates of carbon and nutrient concentrations that could be expected in stormwater runoff exposed to needles and leaves that collect on impervious surfaces. There are six discrepancies between total and dissolved results listed in table 10. There was no evidence of error, and these discrepancies were close to the method detection limit (MDL). Five discrepancies are in P results (range: 0.001 to 0.030 milligrams per liter [mg/L]; MDL 0.010), and one discrepancy of 0.019 mg/L (MDL 0.010) is between total and dissolved N discrepancy in the rain sample (with no leaves added).
Table 10.
Results of analyses of samples of rainwater and rainwater with added mass of pine needles and deciduous leaves collected from leaf-litter solid materials in central and northwestern Vermont.[All concentration values are in milligrams per liter. --, no data]
Total carbon (inorganic + organic), total N, and total P concentrations in bulk precipitation without added organic matter were 0.133, 0.252, and less than 0.010 mg/L, respectively (table 10). In almost all instances, concentrations of all analytes in the samples increased with increasing times that either pine needles or deciduous leaves were soaked in the rainwater. Exceptions were concentrations of total carbon and total particulate P in samples in which leaves were soaked for 2 or 22 hours. To compare these few experimental samples to local environmental data, concentrations of total P in water samples collected from Englesby Brook by Medalie (2007, 2012) between 1999 and 2009 ranged from 0.019 to 11.9 mg/L and averaged about 0.367 mg/L.
Potential Reductions in Phosphorus From Leaf Litter Management
The potential for reduction in total P in stormwater runoff that could be achieved by increasing the cleaning frequency of catch basins and streets to manage leaf litter were investigated by using two approaches: (1) the application of the load-reduction crediting approach developed by the Wisconsin Department of Natural Resources (WDNR, 2022) to conditions in central and northwestern Vermont, using current (2018) municipal BMPs and tree-cover density information; and (2) continuous simulation of stormwater runoff and loads of suspended sediment and total P in a small urban catchment in northwestern Vermont, using WinSLAMM (1996 to 2018; Pitt and Voorhees, 2000; Pitt, 2008). Results of these efforts are intended to support the development of long-term load-reduction credits by the State of Vermont.
Estimated Load-Reduction Credits
Communities that regulate water resources and public works may choose a crediting system to promote techniques designed to reduce constituent loading to waterways, improve receiving water quality, and meet regulatory criteria. Communities that achieve a decrease in loading associated with selected BMPs or SCMs could be awarded credit(s) that serve as a financial incentive for the communities to achieve water-quality goals by using more cost-effective methods.
Load-reduction credits resulting from increased cleaning frequency of catch basins and streets to manage leaf litter were estimated for the seven cooperating MS4 communities and two nonregulated communities by the VTDEC and the University of Vermont. These estimated P-load-reduction credits were used to direct long-term load-reduction efforts and to plan for compliance with regulatory requirements, such as the pollution control plan that had to be developed by each MS4 community.
The WDNR Municipal Phosphorus Reduction Credit for Leaf Management (WDNR, 2022; referred to here as the WI method) was applied to drainage areas within the seven MS4 communities defined by the Lake Champlain Basin SWAT model (Tetra Tech, 2015). The WI method of calculating credits is based on the work by Selbig (2016) and is applied only to medium-density residential land-use areas (MDRAs) with curb-and-gutter drainage systems but without alleys, unless the alleyways receive the same level of leaf collection as streets. Runoff from untreated alleyways may introduce constituent loading in stormwater runoff similar to that from a typical street. Other requirements of the WI method are (1) that the area must have a tree canopy of 17 percent or greater (equivalent to an average of one or more mature trees between the sidewalk and the curb, or within 15 feet of the curb, for every 80 linear feet of curb), (2) a municipal ordinance that prohibits leaf placement in the street, (3) municipal leaf collection at least four times (spaced as conditions require) during October and November, (4) no leaf piles are left on streets overnight, and (5) streets are cleaned within 24 hours of leaf collection.
Estimated P loadings from the drainage basins defined in the Lake Champlain Basin SWAT model were used to develop TMDL target P-load reductions for municipal roads within those basins (Tetra Tech, 2015; EPA, 2016). Target P-load reductions based on existing MS4 credits for CB and SC activities in Massachusetts (2016) were used in concert with land-cover and tree-cover data for the seven MS4 communities in this study, which were available from the Vermont Center for Geographic Information (University of Vermont Spatial Analysis Laboratory, 2019). These data were used to estimate phosphorus load-reduction credits for CB cleaning under two conditions: (1) current CB practices and (2) increased frequency of CB practices (twice per year; table 11). Estimated load-reduction credits for SC practices were also evaluated under four conditions: (1) current SC practices, (2) WI method SC practices applied to MDRAs only, (3) combined current and WI-method SC practices applied to MDRAs only, and (4) WI method applied to all existing SC routes with greater than 17 percent tree cover on average (table 12). Some MS4 communities in the study area implemented BMPs during the TMDL monitoring period (2000 to 2009); if these practices would otherwise qualify for additional credits as part of this exercise, they were prorated at −10 percent per year (tables 11 and 12). For example, the current CB cleaning practices in the SWAT Burlington Bay direct drainage area in the city of Burlington occurs about once every 5 years (table 11), which results in about 0.83 percent total P removed of the TMDL target of 35.7 kilograms per year (kg/yr) for that drainage area. Because this CB cleaning practice was implemented for only a single year during the TMDL monitoring period (2009), the 0.83 percent P reduction goal is prorated for only 1 year, or −10 percent, which shows the current CB cleaning frequency of about every 5 years is meeting about 0.74 percent of the targeted reduction (table 11).
Table 11.
Load-reduction credits estimated for catch-basin cleaning practices conducted by seven MS4 municipalities in northwestern Vermont.[Information courtesy of the Vermont Department of Environmental Conservation and the University of Vermont’s Department of Environmental Studies, Environmental Assessment and Analysis, Service Learning Project, ENSC 202. MS4, municipal separate storm sewer system; SWAT, Soil Water Assessment Tool; TMDL, total maximum daily load; P, phosphorus; kg/yr, kilogram per year; yr, year; CB, catch basin; DD, direct drainage]
Table 12.
Summary of load-reduction credits estimated for street cleaning and street cleaning with leaf management practices conducted by seven MS4 municipalities in northwestern Vermont.[Information courtesy of the Vermont Department of Environmental Conservation and the University of Vermont’s Department of Environmental Studies, Environmental Assessment and Analysis, Service Learning Project, ENSC 202. MS4, municipal separate storm sewer system; SWAT, Soil Water Assessment Tool; TMDL, total maximum daily load; P, phosphorus; kg/yr, kilogram per year; CB, catch basin; SC, street cleaning; WI, Wisconsin, MDRA, medium-density residential area; CB, catch basin; SC, street cleaning; DD, direct drainage]
Maximum P-load-reduction credits attributed to CB cleaning for each of the MS4 communities ranged from 0.01 to 3.4 kg/yr and indicated there was not much potential for increasing CB cleaning credits except for the Saint Albans City CB cleaning program (table 11), which does not currently use a creditable practice. But if Saint Albans City adopted the most effective practice of semiannual CB cleaning, the full credit of 1.36 kg/yr total P removed could be awarded. Conversely, the current SC practices in each community (prorated for the TMDL monitoring period) would result in load-reduction credits ranging from 0.10 to 37 percent, which increased slightly when combined with current CB credits (tables 11 and 12). When evaluating SC as a means to manage leaf litter and applying the WI method strictly to MDRAs, load-reduction credits showed another small increase and ranged from 0 to 51 percent of target reduction (table 12). Combining current SC credits with the WI method applied only to MDRAs further increased the maximum credit to 56 percent of targeted reduction. This application of the WI method, however, requires that existing municipal street-cleaning routes be modified to concentrate in MDRAs with greater than 17 percent tree-cover density. A more pragmatic approach was considered based on the linear relation between leaf-area cover and dissolved phosphorus loading (Janke and others, 2017), and it involved ranking existing MS4 community street-cleaning routes by tree-cover density. By applying the WI method and increasing SC frequency on those routes with tree-cover densities greater than 17 percent, the potential for P-load-reduction credits was considered high for nearly all areas, and the maximum credit increased to 125 percent of the target-load reduction for individual SWAT drainages, with an average increase of over 28 percent for most municipalities (table 12). A USGS data release provides greater details of the estimated P-load-reduction credits attributed to SC within each of the seven MS4 communities in this study (Sorenson and others, 2024).
Simulated Load Reductions
The WinSLAMM model used for this study was developed by the EPA in the 1970s to assess the effects of SC on the quality of stormwater runoff, and its capabilities were expanded through the 1980s as part of the EPA’s National Urban Runoff Program (EPA, 1983). The model was modified for Windows in the 1990s and continues to be updated. Version 10.4.0 of WinSLAMM (1996 to 2018; Pitt and Voorhees, 2000; Pitt, 2008) is capable of simulating stormwater runoff volume, loads of suspended sediments and other constituents, and the effects of many types of structural and nonstructural BMPs/SCMs. A more detailed functional description of the model is provided by Pitt (2008), Garn and others (2010), and Sorenson (2013). Version 10.4.0 contains several major improvements compared to earlier versions, but P removal associated with SC practices in particular have not been fully calibrated and may have a low bias (Pitt, 2008). All input and output files used in the following calibration and simulations are available in Sorenson and others (2024).
Englesby Brook Basin Base Model Development
Englesby Brook is a partly urbanized basin in Burlington and South Burlington Vermont that drains into Lake Champlain. For this study, total annual loads of filterable and total solids are parameters estimated by WinSLAMM and are considered comparable to dissolved and total solids. The difference between filterable and total solids, as defined by the model, therefore may be comparable to particulate solid concentrations, or SSCs. Concentrations and loads of filterable, particulate, and total P were estimated by the WinSLAMM model on the basis of drainage-control and land-use information from State and municipal sources.
More than 55 percent of the 2.41 square kilometer (km2) Englesby Brook Basin is characterized by low- to high-intensity development, followed by about 28 percent developed open space (undeveloped or lightly developed for uses other than agriculture), and the remaining areas are a mix of wetland, forest, and other pervious land-use types (table 1). Figure 8 presents a different perspective of these land-use types within the Englesby Brook Basin, highlighting key impervious runoff source areas, such as roads, other paved areas, and building rooftops interspersed with tree cover and less developed areas such as grass, shrubs and bare soil. About 13 percent of the basin runoff is routed directly to a wastewater treatment facility via a combined sewer system and was not included in the WinSLAMM simulation. This reduction in drainage basin area, plus the small area downstream of the USGS flow and water-quality monitoring site, reduces the effective Englesby Brook drainage area to about 2.10 km2. Figure 9 shows additional details, including the effective drainage area of the Englesby Brook Basin, stormwater inlets, rooftop areas, and street-cleaning frequencies.
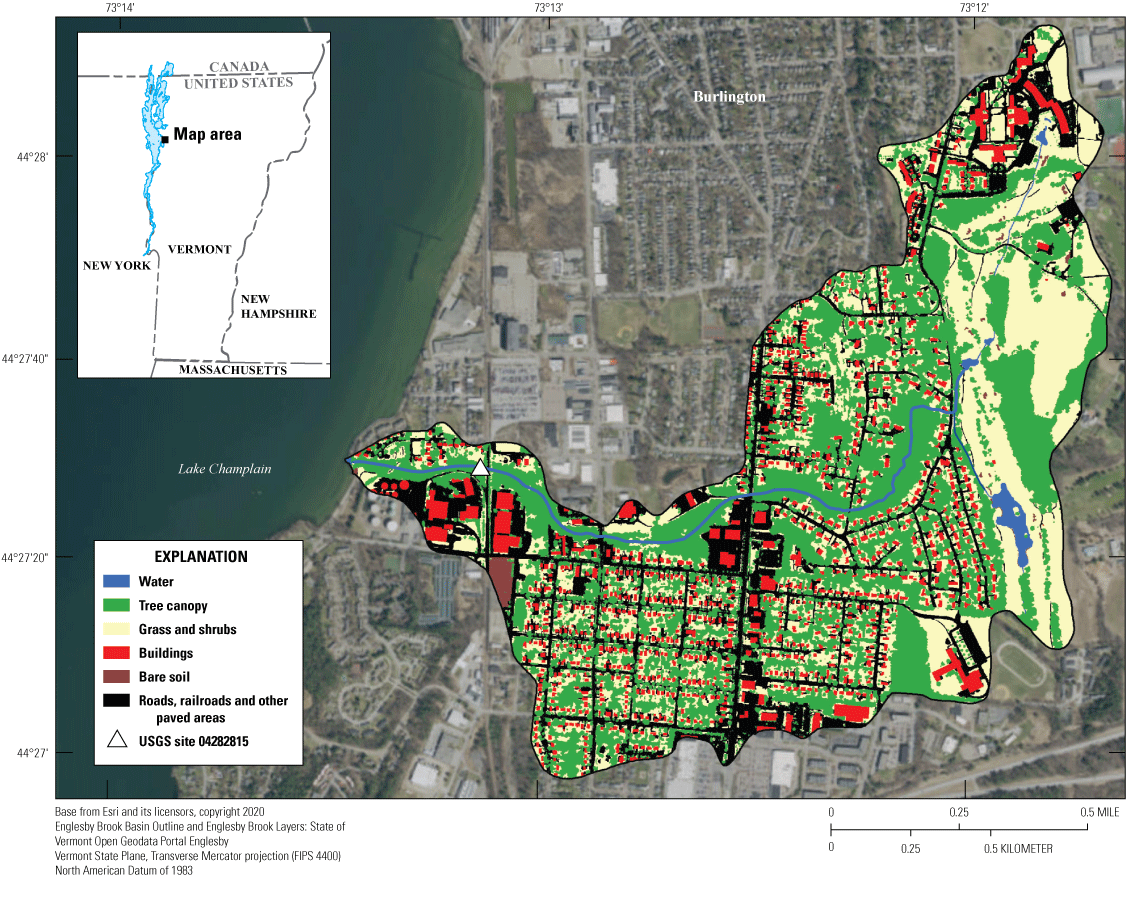
Location map of Englesby Brook Basin showing major land-use types, runoff source areas, and location of the U.S. Geological Survey (USGS) flow and water-quality monitoring site (USGS identifier: 04282815) draining portions of Burlington and South Burlington, Vermont.
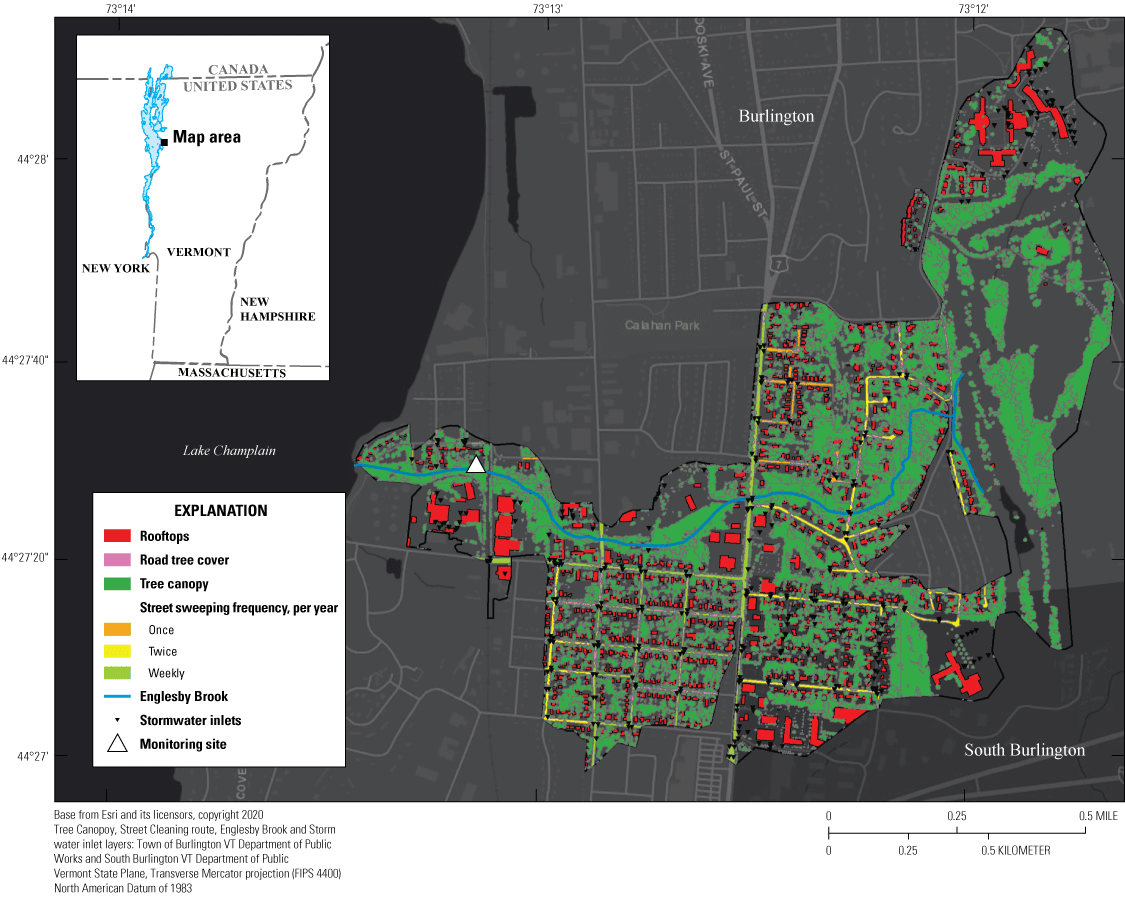
Map of Englesby Brook Basin excluding portions of the basin that drain to combined sewer systems for treatment. Also shown are tree cover, major runoff source areas, and street-cleaning frequency in portions of Burlington and South Burlington, Vermont.
Rainfall data from the Burlington International Airport in South Burlington (National Weather Service, National Climatic Data Center, 2019) and flow data from the Englesby Brook streamgaging station (USGS identifier: 04282815) from 1999 through 2010 were used for comparison with the SLAMM simulation. Because WinSLAMM does not have a groundwater component, base flow was separated from stormflow by a straight line and semilog method (Chapman, 1999; Dingman, 2002; Wittenberg, 2003; Blume and others, 2007), which allows direct comparisons between measured runoff and simulated runoff.
Flow data from Englesby Brook from 1999 and 2000 that were used to calibrate the WinSLAMM model included storm volumes that were equivalent to runoff that ranged from 0.025 to 2.61 cm. Twenty-seven runoff volume observations between 1999 and 2000 were converted to centimeters of runoff by dividing runoff volumes by the effective drainage area of Englesby Brook and used to evaluate the accuracy of the simulated runoff. Runoff coefficients, calculated as the percentage of observed in relation to simulated runoff, were used to refine simulated runoff. Runoff coefficients were used to modify simulated runoff in a stepwise manner, beginning with coefficients for impervious source areas that contribute the greatest relative proportion of total runoff, such as that washing off rooftops. Runoff was calibrated on the basis of a combination of parameter files provided with WinSLAMM, input files adjusted to approximate Englesby Brook flow data, and 1999–2000 precipitation data from Burlington International Airport (Sorenson and others, 2024). The resulting error between the mean depths of simulated and observed runoff was underpredicting by about −5.70 percent, and the sum of the percent differences was about −154 percent. If results from three storms with less than 0.25 cm of precipitation were removed from consideration, error in mean depths of runoff for the remaining 24 storms decreased to about −1.10 percent and the sum of the percent differences was about −26.5 percent (fig. 10). On the basis of data for these 24 storms, figure 11 shows a plot of simulated versus observed runoff from Englesby Brook for October and November 1999. Graphs of the relation of runoff depth to precipitation, and runoff coefficients as a function of precipitation, respectively, are shown in figures 12A and 12B.
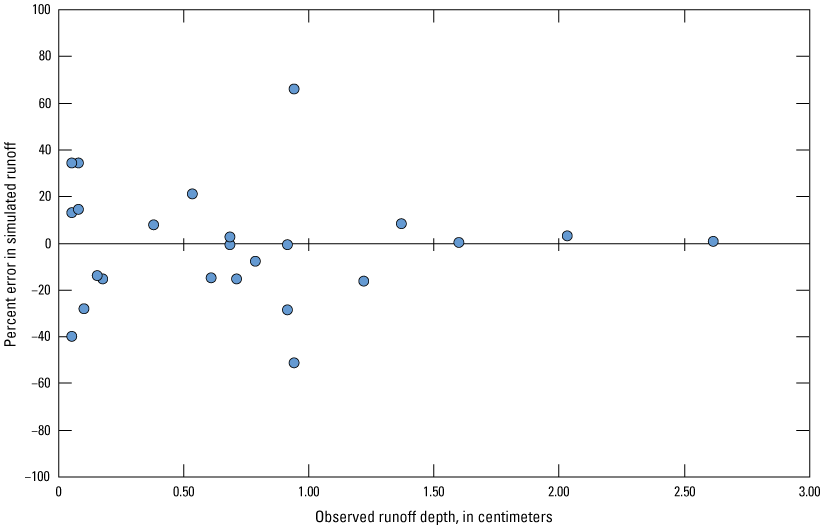
Graph showing percent error of simulated runoff depth as a function of observed runoff depth for precipitation events during 1999 and 2000, at Englesby Brook, Burlington and South Burlington, Vermont (U.S. Geological Survey identifier: 04282815).
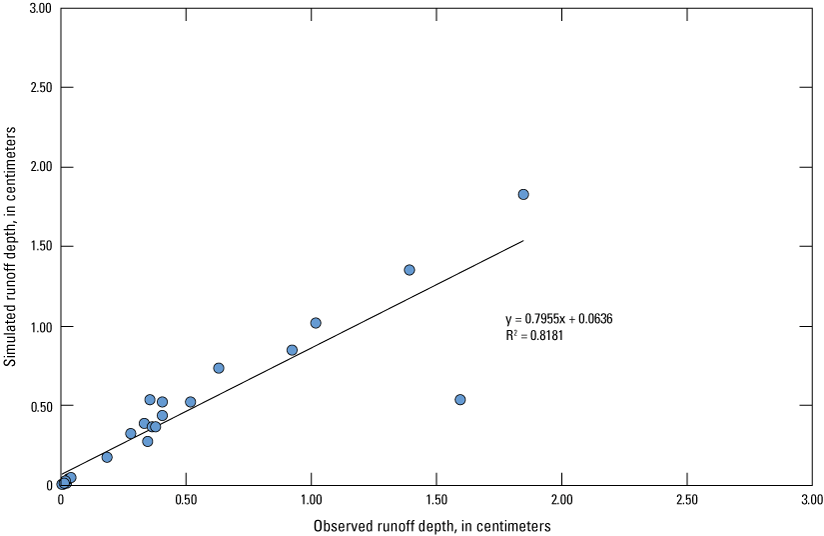
Graph showing simulated runoff depth as a function of observed runoff depth for precipitation events during 1999 and 2000, Englesby Brook, Burlington and South Burlington, Vermont (U.S. Geological Survey identifier: 04282815).
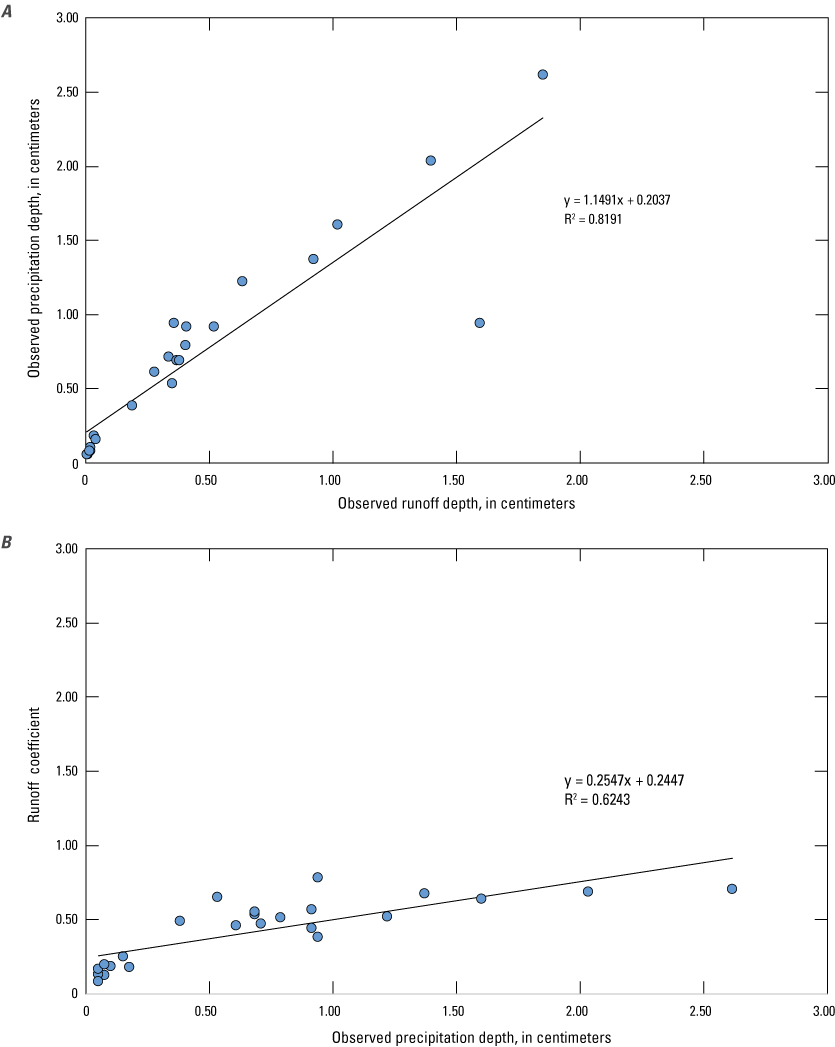
Graphs showing A, observed precipitation as a function of observed runoff depth and B, runoff coefficients as a function of observed precipitation during 1999 and 2000 at Englesby Brook, Burlington and South Burlington, Vermont (U.S. Geological Survey identifier: 04282815).
Concentrations of total N, total P, and suspended sediment concentrations (SSCs) in water samples collected both manually and by automated samplers at the Englesby Brook station (USGS identifier: 04282815) between 1999 and 2009 are summarized in Medalie (2007, 2012) and are available to the public through the National Water Information System (U.S. Geological Survey, 2018).
Some limitations of the data on stormwater flow and constituent concentrations need to be considered as well as limitations associated with the load estimations for Englesby Brook presented in Medalie (2012): (1) the base-flow separation techniques used in this study introduce uncertainty into the final runoff volumes for each storm event used to compare to model performance; (2) water samples were commonly collected at a single fixed depth, which potentially results in a high bias of SSC and other constituents that may be associated with sediment, such as P (Smith, 2002; Bent and others, 2000); and (3) the Load Estimator program (LOADEST; Runkel and others, 2004) used by Medalie (2007) is designed for large streams and is prone to underestimation of loads for smaller, flashy streams (Runkel and others, 2004) such as Englesby Brook. Although Medalie (2007) supplemented LOADEST results with the Graphical Constituent Loading Analysis System (GCLAS) (Koltun and others, 2006) to compensate for the limitations associated with the LOADEST regression approach, some low bias in the Englesby Brook P load results may still be present. Despite these limitations, the flow and water-quality data collected at the Englesby Brook station (USGS identifier: 04282815) presents the opportunity to simulate reductions in loads and concentrations of suspended sediment and P as the result of CB and SC programs within this basin.
Suspended sediment concentrations (referred to as particulate-solid concentrations in WinSLAMM) are multiplied by source area runoff volumes (from streets, for example) by the model and were initially simulated by using a *.pscx file provided with WinSLAMM. Initial results were compared to 13 SSCs from samples collected at Englesby Brook during the 1999 and 2000 calibration period. Values in the initial *.pscx file were then modified in a stepwise manner by adjusting particle solid concentrations associated with each source area, such as roof tops or streets (Sorenson and others, 2024). Mean percent error and the percent difference between the sums of percent error in SSC obtained by using the adjusted *.pscx file were 51 and −667 percent, respectively. For several of the storms, however, concentrations of total P are available for only a single sample or for samples that represent only a portion of the storm hydrograph. Removing two outlier SSC samples reduced the mean percent error and percent difference between the sums of percent error in SSC to about 21 and 233 percent, respectively, for the remaining 11 data points. Pitt (2008) describes WinSLAMM simulations of small urban catchments characterized by multiple land-use types with percent differences between simulated and observed total suspended sediment (TSS) concentrations that ranged between 11 and 66 percent. Although TSS and SSC are not equivalent measures of suspended solids in natural waters (Gray and others, 2000), the comparison of model error metrics indicates that the Englesby Brook model of a natural stream basin draining an urban area with multiple land-use types may have a high bias in estimating highly variable SSC in stormwater runoff.
Total P concentrations simulated by the Englesby Brook base model had a mean percent error between simulated and 55 observed values of about −140 percent, and the percent difference between the sums of simulated and observed total P concentrations was greater than −7,400 percent. Simulations made with a modified *.ppdx file resulted in an improved mean percent error of about −14 percent and a percent difference between sums of the percent error between simulated and observed total P concentrations of about −778 percent. Removal of the same two outlier concentrations, as described above for *.pscx file adjustment to better match Englesby Brook SSC data, improved mean percent error to 0.67 percent and the sums of the differences between observed and simulated results to about 36 percent.
Englesby Brook Basin Model Scenarios
Alternative scenarios were simulated with the calibrated base model to evaluate potential reductions in yields of total solids and total P draining from developed areas within Englesby Brook Basin. Rainfall data from 2010 were used to represent average climatic and runoff conditions based on the average annual flow of 1,860 cubic feet per second at the Winooski River near Essex Junction streamgaging station (USGS identifier: 04290500), which is within 1.9 percent of the 91-year average annual mean flow of 1,820 cubic feet per second at that station. WinSLAMM simulations included the winter period, thus incorporating the effect of the large buildup of materials on street surfaces common during this time, when CB cleaning and SC operations are typically suspended. The three model scenarios were (1) no CB or SC control practices; (2) current (2018) CB and SC operations in which CBs are cleaned every 5 years and the frequency of SC differs by municipal route (weekly, twice per year, and one time per year; fig. 9; tables 2 and 3); and (3) high-frequency control practices, including semiannual CB cleaning and weekly SC to manage leaf loading in the fall.
The respective constituent yields and percent reductions between the no-control scenario and the two-control practice scenarios are shown in table 13. The annual outfall yields of particulate solids and total P under the no-control practice scenario were about 113,000 and 340 kilograms, respectively (table 13). These simulated values are within the ranges of annual loads at the Englesby Brook station (USGS identifier: 04282815) reported by Medalie (2007). Simulated reductions in yields of particulate and total solids were small under current (2018) control practices (2.11 and 1.12 percent, respectively) and increased to 2.73 and 1.42 percent, respectively, under simulated high-frequency control practices. Simulated P reductions also were small. Reductions in yields of particulate P and total P under simulations of current and high-frequency control practices ranged from 0.44 to 0.08 percent, respectively, and from 0.61 to 0.10 percent, respectively, when semiannual CB and weekly SC practices (high-frequency control) were applied. Model scenarios were run with both CB and SC in tandem and separately with similar small reductions.
Table 13.
Simulations of average annual yields of solids and phosphorus from Englesby Brook Basin, which drains portions of the cities of Burlington and South Burlington, Vermont.[Simulations were developed by using the Source Loading and Management Model for Windows (WinSLAMM) program and rainfall data from 2009 and 2010. Comparisons are between noncontrol conditions and two control conditions: (1) current (2018) municipal catch-basin and street-cleaning control practices and (2) high-frequency catch-basin and street-cleaning control practices. kg, kilogram; --, no data; CB, catch basin; SC, street cleaning; 2018 controls, CB cleaning every 5 years and weekly, semi-annual, and to annual SC frequencies; High-frequency controls, semiannual CB cleaning and weekly SC]
The range of simulated street-solid loads and estimated total P content in street-solid loads before and after SC events are listed in table 14. Total P content associated with pre-event and post-event loads was estimated by multiplying simulated street-solid loads by the minimum (0.014 percent) and maximum (0.037 percent) proportions of total P measured in the monthly SC samples collected in 2017 and 2018 (table 8). Minimum total P proportions were between 1.03 and 30.2 percent of street-solid loads and may represent summer street-solid conditions. Maximum total P proportions were between 2.73 and 79.7 percent of street-solid loads and may be representative of the largest street-solid loads seen at the end of winter or in the fall, when large amounts of materials including leaves are present on street surfaces. The maximum percent reduction of street solids attributed to the simulations of weekly street-cleaning control practices was about 29 percent (table 14).
Table 14.
Range of street-solid loads and estimated proportions of total phosphorus simulated for conditions before and after weekly street-cleaning events within the Englesby Brook Basin, which includes portions of the cities of Burlington and South Burlington, Vermont.[Simulations were developed by using the Source Loading and Management Model for Windows (WinSLAMM) program and rainfall data from 2009 and 2010. Proportions of total phosphorus were calculated by using the minimum and maximum monthly proportions in street-cleaning materials listed in table 8. kg, kilogram; kg/curb-mi, kilogram per curb-mile; P, phosphorus]
Summary and Conclusions
The U.S. Geological Survey, in cooperation with the Chittenden County Planning Commission, the Vermont Department of Environmental Conservation, the city of South Burlington, and eight other Vermont municipalities, conducted a study in 2017 and 2018 to evaluate the physical and chemical characteristics of solid materials collected from catch basins and street surfaces. A principal objective of the study was to evaluate the potential reductions in total particulate solids and total phosphorus loading to Lake Champlain receiving waters associated with more intensive street cleaning (SC) during the months of October and November to reduce leaves and other organic materials that contain high concentrations of nutrients.
Composite samples created from catch-basin (CB) and SC solid materials collected by all nine communities were analyzed to provide monthly and seasonal data including the particle-size distribution of the solids and concentrations of total Kjeldahl nitrogen (TKN), total phosphorus (total P), and total organic carbon (TOC) that could be available for runoff to Lake Champlain and other receiving waters.
The distributions of three particle-size fractions in CB and SC solid samples were similar. This similarity between samples agrees with previous work that has shown that catch basins and mechanical-brush street cleaners capture materials within a similar grain-size range, which tends to be coarse (greater than 8 millimeters [mm] in diameter). More than 80 percent of the particles in CB materials were greater than fine sand (0.125 mm in diameter). The finest fraction (less than 0.125 mm in diameter) of solid materials was greatest in the spring of 2018 at nearly 20 percent and was lowest in the fall of 2018 at about 12 percent. Particle-size distributions of SC solid samples were similar to CB distributions, with the medium and coarse fractions comprising 83 to 89 percent of the total materials. SC solids were more representative of seasonal changes in materials, with finer fractions greatest in the spring and summer, whereas in the fall these materials were dominated by leaves and other organic materials.
Concentrations of total Kjeldahl nitrogen, total P, and total organic carbon were greater in SC materials than in CB materials, but concentrations of total P and total organic carbon were similarly distributed in each particle-size fraction. Total Kjeldahl nitrogen concentrations in CB materials were substantially smaller than those in SC except in the finest (less than 0.125 mm in diameter) size fraction. Monthly total Kjeldahl nitrogen concentrations in SC solid samples were two to six times greater than in CB solids. The minimum and maximum concentrations of total P and total organic carbon in monthly samples of SC solids were nearly two times higher than the respective concentrations in CB solids.
Samples of SC solids were collected from materials removed from street surfaces along specific SC routes in two municipalities (Barre and South Burlington) in September and November, 2018. Total P concentrations in samples of street-solid materials collected in November from specific SC routes with greater than 17 percent tree-cover densities were 2 to 30 times greater than in materials collected from the same routes in September, an increase directly attributed to the accumulation of leaves and organic debris on streets in the fall. Estimates of the concentrations of carbon and nutrients that could be expected in rainwater exposed to leaves and organic debris found in the Vermont study area were created by adding known masses of pine needles and deciduous leaves to 2-liter samples of bulk precipitation and allowing them to soak for specific periods of time. The concentration of total P in the deciduous-leaf mixtures represented about 60 percent of the average total P concentration in streamflow reported in studies by the U.S. Geological Survey in Englesby Brook in 1999 and 2009.
Estimated load reductions and associated load-reduction credits resulting from higher frequency CB cleaning and SC to specifically manage leaf litter were developed for the seven cooperating municipal separate storm sewer system communities and two nonregulated communities. Estimates of the maximum reductions in total P loads that could be achieved with CB cleaning practices were small, ranging from 0.01 to only 3.4 kilograms per year, and showed little potential for increasing CB load-reduction credits. Initial estimates of load reductions that could be achieved with current SC practices ranged from 0.10 to 37 percent. When SC practices characterized by the Wisconsin Department of Natural Resources policy medium-density residential areas were applied, estimates of reductions increased up to nearly 56 percent. Applying the Wisconsin Department of Natural Resources policy to all roads with greater than 17 percent tree-cover density increased the estimated maximum range of SC load-reduction credits to about 124 percent of the target-load reduction, with an average increase of more than 28 percent for most communities required to meet federally mandated water-quality guidelines.
The Source Loading and Management Model for Microsoft Windows (WinSLAMM, version 10.4.0) was applied to the 2.41-square-kilometer Englesby Brook Basin in Burlington and South Burlington that drains to Lake Champlain. About 55 percent of the basin area is developed land, about 28 percent is developed open space, and the remaining 17 percent is a mixture of wetland, forest, and other pervious land-use types. Simulation models were calibrated to runoff and water-quality data collected by the U.S. Geological Survey near the mouth of the Englesby Brook Basin in 1999 and 2000. Undeveloped and other pervious areas underlain by silty soils comprised the largest runoff area, but they allow for some infiltration until they become saturated. Water washing off rooftops was the largest source of runoff to the stream from impervious surfaces, followed by that from streets and then other paved surfaces in the basin. The calibrated model was designed to evaluate potential reductions in concentrations of solids (where particulate solids were calibrated to concentrations of suspended sediment) and total P as the result of different control practice scenarios.
Model simulations were calibrated to 2010 climatic conditions, which were determined to be representative of an average year for the study area. Simulations run using 2010 climatic data included the winter period to allow for inclusion of the effect of the large buildup of materials when CB cleaning and SC operations are typically suspended. The three model scenarios were (1) no CB or SC control practices, (2) current (2018) CB and SC operations within the Englesby Brook Basin (CB cleaned every 5 years and route-specific SC: weekly, twice per year, and one time per year), and (3) high-frequency control practices (semiannual CB cleaning and weekly SC). Although the particulate solid concentrations and loads simulated (as suspended sediment concentrations) by the calibrated base model were similar to available flow and water-quality data, simulated reductions of particulate solids (as suspended sediment concentrations) near the mouth of Englesby Brook were small. Simulated reductions in particulate and total solids were small: 2.11 and 1.12 percent, respectively, under current control practices, and 2.73 and 1.42 percent, respectively, when high-frequency controls were applied. Reductions in particulate P and total P from current control practices were also small; between 0.44 and 0.08 percent, respectively. And total P reductions were 0.61 to 0.10 percent, respectively, under the high-frequency control practices scenario. WinSLAMM, however, also estimated about 29 percent reductions in street solids as the result of street cleaning. Applying the proportion of total P that was estimated in Vermont SC materials when leaves are the dominant material being removed from streets, total P represents about 5 to 21 percent of the total mass removed.
Uncertainties in stormwater flow estimates introduced by the hydrograph separation, as well as other assumptions combined with the limitations of the WinSLAMM model to adequately simulate the effect of leaves and organic matter, could explain why increased frequency of CB cleaning and SC collection did not demonstrate larger reductions in solids or concentrations and loads of P near the mouth of Englesby Brook Basin. This study confirms previous work that shows seasonal variations in CB and SC materials with greater masses of nutrients and sediment at the end of winter and fall months. Results of laboratory analyses of rainwater containing leachate from deciduous leaves and pine needles, as well as observations of concentrations of carbon and nutrients in solid material from catch basin and street cleaning in this study, reinforce the findings in previous regional and national studies that these materials, abundant in the fall in Vermont, are considerable sources of carbon, nitrogen, and phosphorus. Furthermore, by intensive removal of leaves and organic materials in the fall, large sources of the dissolved fraction nutrients can be removed from the system and prevented from entering Lake Champlain and other receiving waters. Trees are an essential part of both natural and urban landscapes, but in areas with impervious surfaces that short-circuit natural leaf decomposition processes, combining leaf-removal and street-cleaning practices presents an effective means to minimize excess nutrient and sediment loads delivered by curb-and-gutter systems to receiving waters. An empirical study of the mobility of these types of municipal solid materials during runoff to streams, coupled with controlled applications of best management practices, is an important research opportunity.
References Cited
American Society for Testing and Materials [ASTM], 2019, Standard test methods for laboratory determination of water (moisture) content of soil and rock by mass, West Conshohocken, PA [ASTM–D2216]: ASTM International, [variously paged], accessed September 13, 2017, at https://www.astm.org/d2216-19.html.
Baker, L.A., Kalinosky, P., and Hobbie, S.E., 2014, Quantifying nutrient removal by enhanced street sweeping: Stormwater Magazine, March/April, accessed January 28, 2020, at https://larrybakerlab.cfans.umn.edu/sites/larrybakerlab.cfans.umn.edu/files/files/quantifying-nutrient-removal-compressed-file.pdf.
Bent, G.C., Gray, J.R., Smith, K.P., and Glysson, G.D., 2000, A synopsis of technical issues for monitoring sediment in highway and urban runoff: U.S. Geological Survey Open-File Report 00–497, 51 p., accessed January 17, 2018, at https://doi.org/10.3133/ofr2000497.
Blume, T., Zehe, E., and Bronstert, A., 2007, Rainfall-runoff response, event-based runoff coefficients and hydrograph separation: Hydrological Sciences Journal, v. 52, no. 5, p. 843–862, accessed March 14, 2019, at https://www.tandfonline.com/doi/pdf/10.1623/hysj.52.5.843.
Breault, R.F., Reisig, K.D., Barlow, L.K., and Weiskel, P.K., 2000, Distribution and potential for adverse biological effects of inorganic elements and organic compounds in bottom sediment, Lower Charles River, Massachusetts: U.S. Geological Survey Water-Resources Investigations Report 00–4180, 70 p. [Also available at https://doi.org/10.3133/wri004180.]
Breault, R.F., Sorenson, J.R., and Weiskel, P.K., 2013, Estimated sediment thickness, quality, and toxicity to benthic organisms in selected impoundments in Massachusetts: U.S. Geological Survey Scientific Investigations Report 2012–5191, 42 p., accessed April, 27, 2018, at https://doi.org/10.3133/sir20125191.
Cai, W.-J., Luther, G.W.III, Cornwell, J.C., and Giblin, A.E., 2010, Carbon cycling and the coupling between proton and electron transfer reactions in aquatic sediments in Lake Champlain: Aquatic Geochemistry, v. 16, p. 421–446, accessed March, 21, 2019, at https://link.springer.com/article/10.1007/s10498-010-9097-9.
Chapman, T., 1999, A comparison of algorithms for stream flow recession and baseflow separation: Hydrological Processes, v. 13, no. 5, p. 701–714, accessed March 14, 2019, at https://doi.org/10.1002/(SICI)1099-1085(19990415)13:5<701::AID-HYP774>3.0.CO;2-2.
Colman, J.A., and Sanzolone, R.F., 1991, Surface-water-quality assessment of the Upper Illinois River basin in Illinois, Indiana, and Wisconsin—Geochemical data for fine-fraction streambed sediment from high- and low-order streams, 1987: U.S. Geological Survey Open-File Report 90–571, 108 p. [Also available at https://doi.org/10.3133/ofr90571.]
Cowen, W.F., 1973, Leaves as a source of phosphorus: Environmental Science and Technology, v. 7, no. 9, p. 853–854. [Also available at https://pubs.acs.org/doi/epdf/10.1021/es60081a006.]
Eaton, A.D., Clesceri, L.S., Greenburg, A.E., and Franson, M.A.H., eds., 1998, 4500–NH3 nitrogen (ammonia)—Titrimetric method, in Standard methods for the examination of water and wastewater: Washington, D.C., American Public Health Association, [variously paged], accessed August 10, 2017, at https://www.standardmethods.org/doi/full/10.2105/SMWW.2882.087.
Garn, H.S., Robertson, D.M., Rose, W.J., and Saad, D.A., 2010, Hydrology, water quality, and response to changes in phosphorus loading of Minocqua and Kawaguesaga Lakes, Oneida County, Wisconsin, with special emphasis on effects of urbanization: U.S. Geological Survey Scientific Investigations Report 2010–5196, 54 p., accessed June 20, 2019, at https://doi.org/10.3133/sir20105196.
Gray, J.R., Glysson, G.D., Turcios, L.M., and Schwarz, G.E., 2000, Comparability of suspended-sediment concentration and total suspended solids data: U.S. Geological Survey Water-Resources Investigations Report 2000–4191, 14 p. [Also available at https://doi.org/10.3133/wri004191.]
Hobbie, S.E., Baker, L.A., Buyarski, C., Nidzgorski, D., and Finlay, J.C., 2013, Decomposition of tree leaf litter on pavement—Implications for urban water quality: Urban Ecosystems, no. 17, p. 369–385, accessed January 22, 2018, at https://doi.org/10.1007/s11252-013-0329-9.
Hobbie, S.E., Finlay, J.C., Janke, B.D., Nidzgorski, D.A., Millet, D.B., and Baker, L.A., 2017, Contrasting nitrogen and phosphorus budgets in urban watershed and implications for managing urban water pollution: Proceedings of the National Academy of Sciences of the United States of America, v. 114, no. 16, p. 4177–4182, accessed January 22, 2018, at https://doi.org/10.1073/pnas.1618536114.
Horsley Whitten Group, 2011, Sustainable stormwater funding evaluation for the Upper Charles River communities of Bellingham, Franklin, and Milford, MA: Final report to the U.S. EPA Region I in partnership with AMEC Earth & Environmental, Inc., 174 p., accessed February 3, 2019, at https://www3.epa.gov/region1/npdes/charlesriver/pdfs/20110930-SWUtilityReport.pdf.
Janke, B.D., Finlay, J.C., and Hobbie, S.E., 2017, Trees and streets as drivers of urban stormwater nutrient pollution: Environmental Science and Technology, v. 51, no. 17, p. 9569–9579. [Also available at https://doi.org/10.1021/acs.est.7b02225.]
Koltun, G.F., Eberle, M., Gray, J.R., and Glysson, G.D., 2006, User’s manual for the Graphical Constituent Loading Analysis System (GCLAS): U.S. Geological Survey Techniques and Methods, book 4, chap. C1, 51 p., accessed January 20, 2020, at https://www.usgs.gov/software/gclas-graphical-constituent-loading-analysis-system-a-tool-computing-loads-constituents.
Lipps W.C., Baxter, T.E., and Braun-Howland, E., eds., 2018a, 4500–P F. Phosphorus—Automated ascorbic acid reduction method, in Standard methods for the examination of water and wastewater: Washington, D.C., American Public Health Association Press, [variously paged], accessed August 10, 2018, at https://www.standardmethods.org/doi/full/10.2105/SMWW.2882.093.
Lipps, W.C., Baxter, T.E., and Braun-Howland, E., eds., 2018b, 5310B Total organic carbon—High-temperature combustion method, in Standard methods for the examination of water and wastewater: Washington, D.C., American Public Health Association Press, accessed February 13, 2021, at https://www.standardmethods.org/doi/10.2105/smww.2882.104.
Martialay, M.L., 2015, Role of fresh water lakes, reservoirs in the global carbon cycle: ScienceDaily, September 3, 2015, Rensselaer Polytechnic Institute (RPI), accessed March 16, 2018, at https://www.sciencedaily.com/releases/2015/09/150903160644.htm.
Massachusetts Department of Environmental Protection, 2016, General Permit for stormwater discharges from small municipal separate storm sewer systems in Massachusetts: U.S. Environmental Protection Agency, 61 p., accessed March 18, 2018, at https://www3.epa.gov/region1/npdes/stormwater/ma/2016fpd/final-2016-ma-sms4-gp-mod.pdf.
Medalie, L., 2007, Concentrations and loads of nutrients and suspended sediments in Englesby Brook and Little Otter Creek, Lake Champlain Basin, Vermont, 2000–2005: U.S. Geological Survey Scientific Investigations Report 2007–5074, 50 p., accessed March 14, 2018, at https://doi.org/10.3133/sir20075074.
Medalie, L., 2012, Effects of urban best management practices on streamflow and phosphorus and suspended sediment transport on Englesby Brook in Burlington, Vermont, 2000–2010: U.S. Geological Survey Scientific Investigations Report 2012–5103, 26 p., accessed March 14, 2018, at https://pubs.usgs.gov/sir/2012/5103/.
National Weather Service, National Climatic Data Center, 2019, Precipitation climatology for Burlington International Airport, VT, Coop ID 431081: National Oceanic and Atmospheric Administration data, accessed May 5, 2019, at https://www.ncdc.noaa.gov/cdo-web/datasets/GHCND/stations/GHCND:USW00014742/detail.
Office for Coastal Management, 2020, NOAA's Coastal Change Analysis Program (C-CAP) 2006 regional land cover data—Coastal United States: National Oceanic and Atmospheric Administration dataset, accessed 2020 at https://www.fisheries.noaa.gov/inport/item/48333.
Patton, C.J., and Kryskalla, J.R., 2003, Methods of analysis by the U.S. Geological Survey National Water Quality Laboratory—Evaluation of alkaline persulfate digestion as an alternative to Kjeldahl digestion for determination of total and dissolved nitrogen and phosphorus in water: U.S. Geological Survey Water-Resources Investigations Report 2003–4174, 33 p. [Also available at https://doi.org/10.3133/wri034174.]
Patton, C.J., and Truitt, E.P., 2000, Methods of analysis by the U.S. Geological Survey National Water Quality Laboratory—Determination of ammonium plus organic nitrogen by a Kjeldahl digestion method and an automated photometric finish that includes digest cleanup by gas diffusion: U.S. Geological Survey Open-File Report 2000–170, 31 p., accessed March 14, 2018, at https://doi.org/10.3133/ofr00170.
Runkel, R.L., Crawford, C.G., and Cohn, T.A., 2004, Load Estimator (LOADEST)—A FORTRAN program for estimating constituent loads in streams and rivers: U.S. Geological Survey Techniques and Methods, book 4, chap. A5, 69 p., accessed January 20, 2020, at https://pubs.usgs.gov/tm/2005/tm4A5/pdf/508final.pdf.
Selbig, W.R., 2016, Evaluation of leaf removal as a means to reduce nutrient concentrations and loads in urban stormwater: Science of the Total Environment, v. 571, p. 124–133. [Also available at https://doi.org/10.1016/j.scitotenv.2016.07.003.]
Selbig, W.R., and Bannerman, R.T., 2007, Evaluation of street sweeping as a stormwater-quality-management tool in three residential basins in Madison, Wisconsin: U.S. Geological Survey Scientific Investigations Report 2007–5156, 103 p., accessed January 11, 2008, at https://doi.org/10.3133/sir20075156.
Selbig, W.R., Buer, N.H., Bannerman, R.T., and Gaebler, P., 2020, Reducing leaf litter contributions of phosphorus and nitrogen to urban stormwater through municipal leaf collection and street cleaning practices: U.S. Geological Survey Scientific Investigations Report 2020–5109, 17 p., accessed March 23, 2020, at https://doi.org/10.3133/sir20205109.
Smith, K.P., 2002, Effectiveness of three best management practices for highway-runoff quality along the Southeast Expressway, Boston, Massachusetts: U.S. Geological Survey Water-Resources Investigations Report 02–4059, 170 p. [Also available at https://doi.org/10.3133/wri024059.]
Smith, K.P., 2010, Effectiveness of catch basins equipped with hoods in retaining gross solids and hydrocarbons in highway runoff, Southeast Expressway, Boston, Massachusetts, 2008–09: U.S. Geological Survey Scientific Investigations Report 2010–5182, 23 p., accessed March 5, 2017, at https://pubs.usgs.gov/sir/2010/5182.
Smith, K.P. and Granato, G.E., 2010, Quality of stormwater runoff discharged from impervious surfaces of Massachusetts highways, 2005–2007: U.S. Geological Survey Scientific Investigations Report 2009–5269, 198 p. [Also available at https://doi.org/10.3133/sir20095269.]
Sorenson, J.R., 2013, Potential reductions of street solids and phosphorus in urban watersheds from street cleaning, Cambridge, Massachusetts, 2009–11: U.S. Geological Survey Scientific Investigations Report 2012–5292, 66 p., plus appendix 1 on a CD–ROM in pocket. [Also available at https://doi.org/10.3133/sir20125292.]
Sorenson, J.R., Pease, J.M., Foote, J.K., Chalmers, A.T., Ainley, D.H., and Williams, C.J., 2024, Data supporting phosphorus load-reduction estimates from leaf-litter removal in central and northwestern Vermont: U.S. Geological Survey data release, https://doi.org/10.5066/P9A44LIX.
Tetra Tech, 2015, Lake Champlain Basin SWAT model configuration, calibration and validation: Prepared for the U.S. Environmental Protection Agency, 52 p., accessed December 28, 2018, at https://www.epa.gov/sites/default/files/2015-09/documents/swat-model-configuration-calibration-validation.pdf.
Tetra Tech, 2016, BMP Accounting & Tracking Tool (BATT): Prepared for the U.S. Environmental Protection Agency, Region 1, 34 p., accessed December 28, 2018, at https://www3.epa.gov/region1/npdes/stormwater/ma/batt-users-guide.pdf.
University of Vermont Spatial Analysis Laboratory, 2019, LandLandcov_TreeCanopy2016: Vermont Center for Geographic Information dataset, accessed 2019 at https://maps.vcgi.vermont.gov/gisdata/metadata/LandLandcov_TreeCanopy2016.htm.
U.S. Department of Agriculture, Natural Resources Conservation Service, 2009, Hydrologic soil groups, chap. 7 of National engineering handbook, part 630 hydrology: U.S. Department of Agriculture, 13 p., accessed January 10, 2019, at https://directives.sc.egov.usda.gov/OpenNonWebContent.aspx?content=22526.wba.
U.S. Environmental Protection Agency [EPA], 1974, (Method 415.1)—Organic carbon, total (combustion or oxidation) in drinking, surface and saline waters, domestic and industrial wastes (rev. 1): U.S. Environmental Protection Agency, 3 p. accessed January 10, 2019, at https://settek.com/documents/EPA-Methods/PDF/EPA-Method-4151.pdf.
U.S. Environmental Protection Agency [EPA], 1983, Results of the nationwide urban runoff program, Volume 1—Final report: U.S. Environmental Protection Agency, EPA–WH 554, 198 p., accessed November 14, 2020, at https://www3.epa.gov/npdes/pubs/sw_nurp_vol_1_finalreport.pdf.
U.S. Environmental Protection Agency [EPA], 1993, Method 351.2, revision 2.0—Determination of total Kjeldahl nitrogen by semi-automated colorimetry: U.S. Environmental Protection Agency, 15 p., accessed November 14, 2020, at https://www.epa.gov/sites/default/files/2015-08/documents/method_351-2_1993.pdf#:~:text=www.epa.gov%20August%201993%20Method%20351.2%2C%20Revision%202 .0%3A%20Determination,Total%20Kjeldahl%20Nitrogen%20by%20Semi-Automated%20Colorimetry%20METHOD%20351.2.
U.S. Environmental Protection Agency [EPA], 2004, Method 9060A—Total organic carbon (rev. 1, November 2004): U.S. Environmental Protection Agency, 5 p. accessed January 10, 2019, at https://www.epa.gov/sites/default/files/2015-12/documents/9060a.pdf.
U.S. Geological Survey, 2018, USGS Englesby Brook at Burlington, VT in USGS water data for the Nation: U.S. Geological Survey National Water Information System database, accessed March 27, 2018, at https://doi.org/10.5066/F7P55KJN. [Site information directly accessible at https://waterdata.usgs.gov/nwis/dv?referred_module=sw&site_no=04282815.]
Wallace, T.A., Ganf, G.C., and Brokkes, J.D., 2008, A comparison of phosphorus and DOC leachates from different types of leaf litter in an urban environment: Freshwater Biology, v. 53, p. 1902–1913. [Also available at https://doi.org/10.1111/j.1365-2427.2008.02006.x.]
Waschbusch, R.J., Selbig, W.R., and Bannerman, R.T., 1999, Sources of phosphorus in stormwater and street dirt from two urban residential basins in Madison, Wisconsin, 1994–95: U.S. Geological Survey Water-Resources Investigations Report 99–4021, 47 p. [Also available at https://doi.org/10.3133/wri994021.]
Wisconsin Department of Natural Resources [WDNR], 2022, Municipal phosphorus reduction credit for leaf management programs, February 17, 2022, EGAD Number: 3800-2022-01: Wisconsin Department of Natural Resources, 11 p, March 2022 at https://dnr.wisconsin.gov/sites/default/files/topic/Stormwater/LeafManagementCredit.pdf.
Wittenberg, H., 2003, Effects of season and man-made changes on baseflow and flow recession—Case studies: Hydrological Processes, v. 17, no. 11, p. 2113–2123, accessed March 14, 2019, at https://doi.org/10.1002/hyp.1324.
Wright, S.F., 2003, Glacial geology of the Burlington and Colchester 7.5’ quadrangles, northern Vermont: Vermont Department of Environmental Conservation, 12 p., accessed December 27, 2017, at https://dec.vermont.gov/sites/dec/files/geo/documents/GlacGeoBurlwright.pdf.
Conversion Factors
International System of Units to U.S. customary units
Multiply | By | To obtain |
---|---|---|
micrometer (µm) | 0.00003937 | inch (in.) |
millimeter (mm) | 0.03937 | inch (in.) |
milliliter (mL) | 0.03381 | ounce, fluid (fl. oz) |
liter (L) | 0.2642 | gallon (gal) |
gram (g) | 0.03527 | ounce, avoirdupois (oz) |
kilogram (kg) | 2.205 | pound, avoirdupois (lb) |
kilogram per year (kg/yr) | 2.205 | pound per year (lb/yr) |
Temperature in degrees Celsius (°C) may be converted to degrees Fahrenheit as follows:
°F = (1.8 × °C) + 32.
Temperature in degrees Fahrenheit (°F) may be converted to degrees Celsius (°C) as follows:
°C = (°F – 32) / 1.8.
Supplemental Information
Specific conductance is given in microsiemens per centimeter at 25 degrees Celsius (µS/cm at 25 °C).
Concentrations of chemical constituents in water are given in milligrams per liter (mg/L). Concentrations of chemical constituents in solids are given in either milligrams per kilogram (mg/kg) or micrograms per gram (µg/g).
Abbreviations
BMP
best management practice
CB
catch basin
DIW
deionized water
EPA
U.S. Environmental Protection Agency
FRP
flow restoration plan
HCl
hydrochlorid acid solution
LOADEST
Load Estimator
MDL
method detection limit
MDRA
medium-density residential land-use area
MS4
municipal separate storm sewer system
N
nitrogen
NWQL
National Water Quality Laboratory
P
phosphorus
RPD
relative percent difference
SC
street cleaning
SCM
stormwater control measure
SSC
suspended sediment concentration
SWAT
Soil Water Assessment Tool
TKN
total Kjeldahl nitrogen
TMDL
total maximum daily load
TOC
total organic carbon
TSS
total suspended sediment
USGS
U.S. Geological Survey
VTDEC
Vermont Department of Environmental Conservation
WDNR
Wisconsin Department of Natural Resources
WI method
WDNR Municipal Phosphorus Reduction Credit for Leaf Management
WinSLAMM
Source Loading and Management Model for Windows
For more information about this report, contact:
Director, New England Water Science Center
U.S. Geological Survey
10 Bearfoot Road
Northborough, MA 01532
dc_nweng@usgs.gov
or visit our website at
https://www.usgs.gov/centers/new-england-water
Publishing support provided by the Pembroke, Moffett Field, and Sacramento Publishing Service Centers
Disclaimers
Any use of trade, firm, or product names is for descriptive purposes only and does not imply endorsement by the U.S. Government.
Although this information product, for the most part, is in the public domain, it also may contain copyrighted materials as noted in the text. Permission to reproduce copyrighted items must be secured from the copyright owner.
Suggested Citation
Sorenson, J.R., Pease, J.M., Foote, J.K., Chalmers, A.T., Ainley, D.H., and Williams, C.J., 2024, Estimated reductions in phosphorus loads from removal of leaf litter in the Lake Champlain drainage area, Vermont: U.S. Geological Survey Scientific Investigations Report 2023–5104, 46 p., https://doi.org/10.3133/sir20235104.
ISSN: 2328-0328 (online)
Study Area
Publication type | Report |
---|---|
Publication Subtype | USGS Numbered Series |
Title | Estimated reductions in phosphorus loads from removal of leaf litter in the Lake Champlain drainage area, Vermont |
Series title | Scientific Investigations Report |
Series number | 2023-5104 |
DOI | 10.3133/sir20235104 |
Year Published | 2024 |
Language | English |
Publisher | U.S. Geological Survey |
Publisher location | Reston, VA |
Contributing office(s) | New England Water Science Center |
Description | Report: viii, 46 p.; Data Release |
Country | United States |
State | Vermont |
Online Only (Y/N) | Y |
Additional Online Files (Y/N) | N |