Groundwater and Surface-Water Interactions in the Lower Duwamish Waterway, Seattle, Washington
Links
- Document: Report (9.6 MB pdf) , HTML , XML
- Data Release: USGS data release - Data in support of groundwater- and surface-water-interactions report— Groundwater and surface-water interactions in the Lower Duwamish Waterway, Seattle, Washington
- Download citation as: RIS | Dublin Core
Abstract
The U.S. Geological Survey (USGS), in cooperation with the Washington State Department of Ecology (Ecology), conducted a study to describe the current understanding of the regional groundwater system of the lower Duwamish River valley and groundwater and surface-water interactions in the lower Duwamish Waterway. The lower Duwamish Waterway is the final 5-mile (mi) reach of the Duwamish River before it empties into Elliott Bay in Puget Sound near Seattle, Washington. A nearshore site (hereinafter referred to as “Nearshore Site” to distinguish the particular site from general discussions of nearshore areas) along the western shoreline of the Duwamish River, about 1.5 mi upstream from the river mouth, was selected for focused groundwater data collection by USGS. Data loggers were deployed in seven groundwater wells and one stilling well in the Duwamish River to measure specific conductance, temperature, and depth at 15-minute intervals for a period of about 2 years.
At the Nearshore Site during 2020–22, water levels in the shallow wells were 3–8 feet (ft) higher than water levels in the deep wells, providing evidence for a low-permeability layer between the shallow and deep aquifers in this area. The shallow wells had a pronounced seasonal variability, with high water levels in winter and low water levels in summer. Data from the deep wells showed far less seasonal variability, with slight increases in winter and a near-constant water level from spring to autumn. The deep wells had a strong hydraulic connection to the Duwamish River, as evidenced by the synchronous water-level variability during the tidal cycle, whereas the shallow wells had minimal to no tidal response. The potentiometric maps developed for the Nearshore Site and surrounding areas indicate large differences in groundwater-flow directions for the shallow and deep aquifers at low and high tides. For the shallow aquifer, flow is toward the lower Duwamish Waterway near the Nearshore Site, regardless of the tidal condition. For the deep aquifer, a potentiometric trough forms parallel to the shoreline during high tide, indicating that groundwater flow converges from the uplands to the west and the Duwamish River to the east. The geometry of the potentiometric surfaces between the nearshore-most well and the shoreline is complex and is further confounded by intermittent shoreline armoring and other buried infrastructure, which could serve as either a barrier or a conduit to flow.
Groundwater and surface-water interactions in the lower Duwamish Waterway are inherently complex as a result of three overarching factors. First, water levels in the lower reaches of the Duwamish River vary daily by 11–16 ft because of tides from Puget Sound, which create large swings in the hydraulic gradient in the nearshore groundwater system. Second, the density and chemical composition of water in the Duwamish River change daily with the tides and seasonally, which constrains how river water entering the nearshore sediments interacts with discharging groundwater. Third, the nearshore subsurface and shoreline conditions are heterogenous because of extensive shoreline armoring over the past century, which governs the flow of groundwater and infiltrating river water. These unique features of groundwater and surface-water interactions in the lower Duwamish Waterway thus govern the transport of terrestrial contaminants to the lower Duwamish Waterway. Furthermore, the heterogenous aquifer properties in the lower Duwamish Waterway contribute to spatially and temporally dynamic contaminant-transport processes.
Introduction
The lower Duwamish Waterway is the final 5-mile (mi) reach of the Duwamish River before it empties into Elliott Bay in Puget Sound near Seattle, Washington (fig. 1). The reach was straightened in the early 1900s and is maintained through regular dredging as a navigable waterway. The adjacent floodplains were filled with dredged river sediment and imported artificial fill material to support numerous industrial uses, including manufacturing, warehousing, and marinas, as well as residential development. Current and historical human activities have contaminated sediments within the waterway and the adjacent groundwater system. During 2001–02, the U.S. Environmental Protection Agency (EPA) and the Washington State Department of Ecology (Ecology) required remedial investigations and feasibility studies on the lower Duwamish Waterway pursuant to the Comprehensive Environmental Response, Compensation, and Liability Act (also known the “Superfund” program; 42 U.S.C 103) and the Washington Model Toxics Control Act (Revised Code of Washington, chapter 70A.305; Washington State Legislature, 1989) because of concerns about human health risks from exposure to contaminated sediments (Lower Duwamish Waterway Group, 2010). The main contaminants of concern for human health include polychlorinated biphenyls (PCBs), dioxins/furans, carcinogenic polycyclic aromatic hydrocarbons (cPAHs), and arsenic. Additionally, about 41 compounds (including individual metals, PCBs, polycyclic aromatic hydrocarbons [PAHs], phthalates, and other semi-volatile organic compounds) have been identified as contaminants of concern for protection of the benthic community (Washington State Department of Ecology, 2013). The EPA final cleanup plan was released in November 2014 and included using combinations of dredging, capping, natural sedimentation, and enhanced natural recovery (U.S. Environmental Protection Agency, 2014).
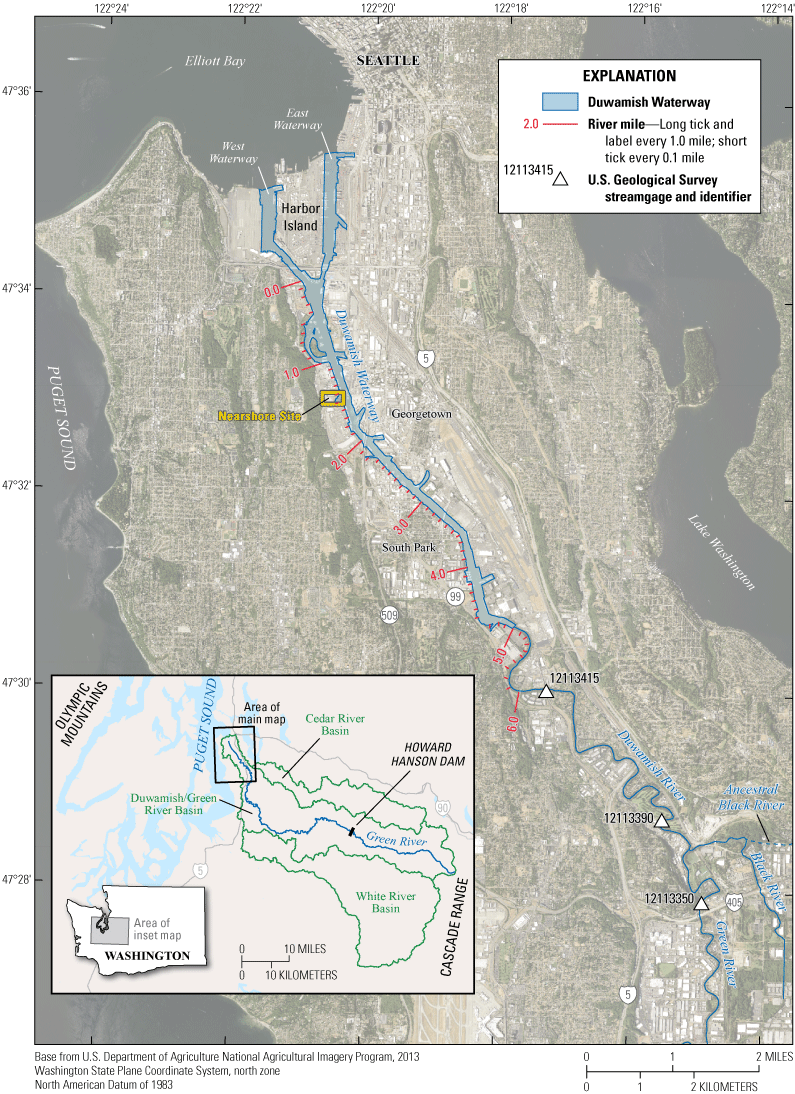
Location of U.S. Geological Survey streamgages, lower Duwamish Waterway, Seattle, Washington.
To support the implementation of a cleanup plan of contaminated sediments in the lower Duwamish Waterway, Ecology is leading source-control activities and a watershed-scale pollutant-loading assessment to identify sources and mechanisms of sediment recontamination adjacent to and upstream from the lower Duwamish Waterway. On-going contaminant sources that have the potential to recontaminate recently remediated lower Duwamish Waterway sediment include upstream sources in the watershed transported to the lower Duwamish Waterway by river flow and sediment (Conn and others, 2018; Senter and others, 2018; King County, 2018), stormwater outfalls (SAIC and Newfields, 2011), and direct contact with nearshore properties within the lower Duwamish Waterway (Washington State Department of Ecology, 2023a). One of the ways Ecology regulates contamination to surface water and sediments is by conducting cleanups of terrestrial sites adjacent to the lower Duwamish Waterway, including contaminated soil and groundwater that have the potential to migrate into the surface water and sediments. These terrestrial areas adjacent to the lower Duwamish Waterway are referred to, in the context of Ecology source control and cleanup activities, as “uplands.” In this report, these areas are described as terrestrial areas adjacent to the lower Duwamish Waterway, or nearshore areas. The term “uplands” in this report is used in a broader geographic context within the Duwamish River valley.
Ecology manages the cleanup of numerous contaminated nearshore areas on a site-by-site basis within 24 designated lower Duwamish Waterway source-control areas (Washington State Department of Ecology, 2023a). The nature and extent of contamination varies by site, and can include soil, groundwater, surface water, air, sediment, and bedrock matrices for a range of contaminants including heavy metals, organic chemicals, petroleum products, and others. Summaries of groundwater chemistry in the lower Duwamish Waterway have been compiled (Leidos, Inc., 2017; Senter and others, 2019), but little is understood about contaminant transport and fluxes to the lower Duwamish Waterway. The locations and fluxes of contaminant discharge to the lower Duwamish Waterway from nearshore areas is influenced by numerous hydrogeochemical factors. Factors include meteorology, subsurface characteristics, location and concentration of contaminants on the site, chemical properties (for example, water solubility, ionic and nonionic sorption properties), and others.
From bottom to top, the groundwater system in the Duwamish River valley consists of a shallow bedrock trough, interfingering glacial and interglacial unconsolidated sediments (unconsolidated sediments), and recent fill from anthropogenic activities beginning in the 1900s. The primary aquifer(s) for groundwater flow extend from the bottom of the fill units into the underlying unconsolidated sediments. In some places, thin layers of silt and other fine-grained material separate a shallow zone from a deeper zone; however, these layers tend to be discontinuous, and the groundwater system generally behaves as a single aquifer on a regional scale (Booth and Herman, 1998). Groundwater flow is from the uplands toward the river. As groundwater approaches the river, its movement becomes more complex because of varying salinity and stage in the river.
A mixing zone forms at the interface between discharging groundwater and receiving surface water and also extends inland by a few feet to a few tens of feet. The mixing zone is a region of dynamic hydrogeochemical conditions that can impact contaminant concentrations and fluxes, such as changes in redox, temperature, flow paths, and biological activity (for example, see Fritz and Arntzen, 2007). Processes within the mixing zone are further complicated in tidally influenced locations, such as the lower Duwamish Waterway, owing to density differences between the fresh groundwater and saline surface water as well as the changing water level at the receiving water boundary (Li and others, 2003; Robinson and others, 2018). An understanding of the regional hydrogeology is fundamental to assessing contaminant transport processes from nearshore sites to receiving waters.
Four key past studies provided the foundation for understanding the groundwater system in the Duwamish River valley. Woodward and others (1995) conducted an evaluation of groundwater occurrence and quality for southwestern King County, which included the area between the Duwamish River and Puget Sound to the west. A conceptual groundwater model of the Duwamish River valley by Booth and Herman (1998) provided the first groundwater study focused on the Duwamish River valley and was used to inform a numerical groundwater-flow model by Fabritz and others (1998). Finally, the Lower Duwamish Waterway Group (2010) led a remedial investigation to assess the extent of contamination of the lower Duwamish Waterway. Additional studies providing background information are described in section, “Description of the Study Area.”
With the EPA Record of Decision complete for the Superfund site (U.S. Environmental Protection Agency, 2014) and clean-up expected to begin around 2024, there remains a lack of understanding of groundwater and surface-water interactions, especially in the estuarine lower Duwamish Waterway that has adjacent terrestrial sites with known contamination. The Duwamish River is a stratified estuary with a large tidal range that extends many river miles upstream from the lower Duwamish Waterway (see section, “Duwamish River Characteristics”). The characteristics and extent of the lower Duwamish Waterway estuary are continuously fluctuating because of the interaction between upstream fresh water and underlying downstream salt water. Fundamental knowledge gaps regarding groundwater and surface-water interactions in the lower Duwamish Waterway include:
-
• groundwater flow paths from recharge to discharge zones,
-
• the presence and continuity of confining layers,
-
• the inland extent of the freshwater/saltwater interface under varying river conditions, and
-
• the spatial and temporal dynamics of the interaction between surface water and groundwater.
Much of the existing data and understanding are on a site-by-site basis and a regional assessment of groundwater and surface-water interactions is lacking. During 2020–23, the U.S. Geological Survey (USGS), in cooperation with Ecology, compiled existing data and collected new data to improve the understanding of groundwater and surface-water interactions in the lower Duwamish Waterway. The scope included:
-
• compiling existing lower Duwamish Waterway groundwater data,
-
• a detailed assessment of one nearshore site adjacent to the lower Duwamish Waterway, and
-
• the development of a regional conceptual groundwater model by synthesizing existing data with new site-specific data.
This report presents the results of this work to describe the current understanding of the regional groundwater system and groundwater and surface-water interactions in the lower Duwamish Waterway. The information will support ongoing contaminant source-control activities as they relate to subsurface contaminant-transport and contamination-attenuation processes between the contaminated nearshore sites and the lower Duwamish Waterway.
Description of the Study Area
The study area is defined as the lowermost 5 mi of the Duwamish River—often referred to as the lower Duwamish Waterway—and the adjacent lands, collectively called the lower Duwamish River valley (fig. 1). The Duwamish River is about 12-mi long, begins at the confluence of the Black and Green Rivers, and enters Puget Sound at Elliot Bay in Seattle, Washington. The Green River system, which is the primary source of the Duwamish River, originates in the Cascade Range to the east, generally flowing west and northwest. The land adjacent to the Duwamish River is a hub for industry and commercial activities and serves as a major shipping route for cargo. The area is also home to two neighborhoods—South Park and Georgetown—that include a mixture of residential, recreational, commercial, and industrial land uses. A common approach for referencing features along and near the Duwamish River is to use river distances (for example, river mile [RM] 5.0), which are measured from the mouth of the river at an arbitrarily established line drawn across the west waterway near the south end of Harbor Island (fig. 1).
Seattle has a temperate marine climate with mean monthly temperatures ranging from 67 degrees Fahrenheit (°F) in July to 42 °F in December (National Oceanic and Atmospheric Administration, 2023a). The study area has a dry summer season from May to September and a wet winter season from October to April. Mean monthly rainfall ranges from about 6.1 inches (in) in November to about 0.7 in in August (National Oceanic and Atmospheric Administration, 2023a).
The Duwamish River valley is characterized by an extensive floodplain that abuts the Duwamish River and is separated from the adjacent uplands by steep valley walls (fig. 2). From RM 5.0 to the mouth of the river, the width of the floodplain ranges from about 1.0 to 1.75 mi, and the distance to the valley walls is generally greater on the east side of the river. Most of the land in the floodplain is less than about 20 feet (ft) above North American Vertical Datum of 1988 (NAVD 88) (which is near modern [2024] sea level), other than a few conspicuous mounds of exposed bedrock near RM 4.0. The altitude of the upland plains to the east and west of the Duwamish River in the study area exceeds 350 and 500 ft, respectively.
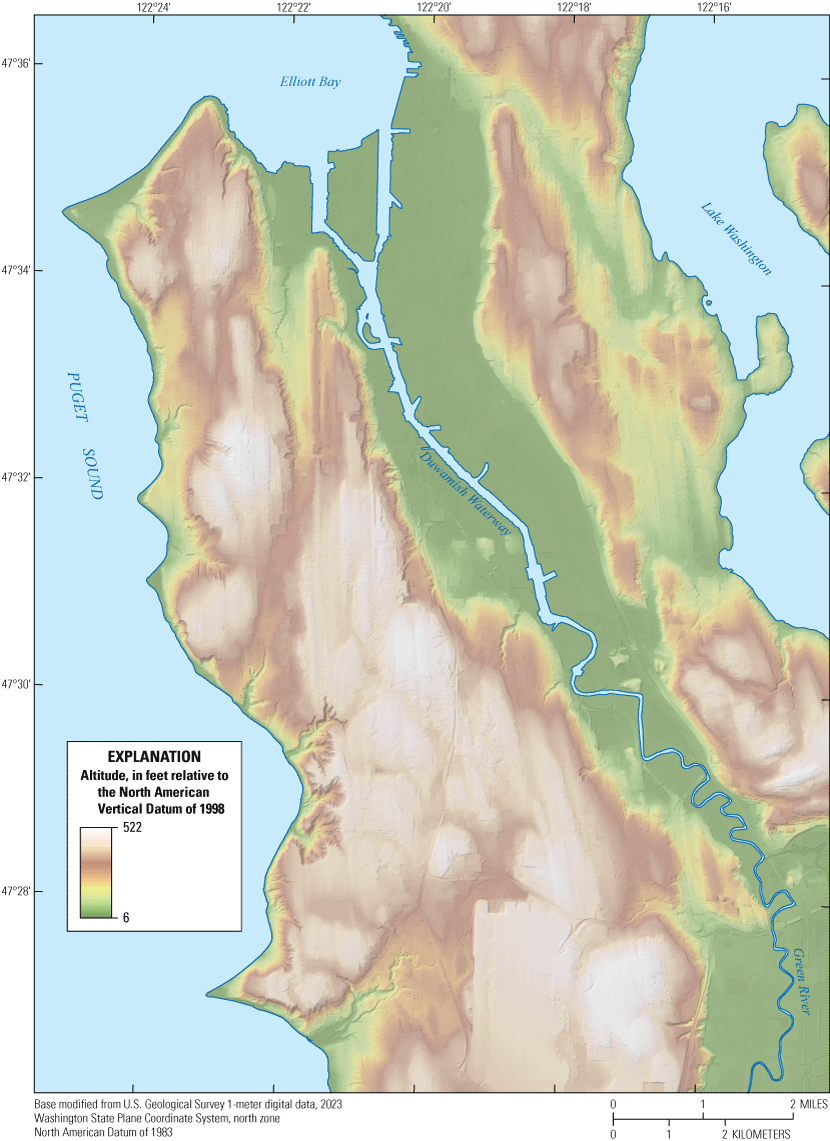
Elevation map of the area surrounding the lower Duwamish Waterway, Seattle, Washington. Digital elevation model has a 1-meter resolution and is from U.S. Geological Survey (2023a).
Land cover in the study area is dominated by development and impervious surfaces. The upland areas contain occasional regions of forest or other vegetation, most prominently along the western valley wall between the mouth of the river and RM 3.0. Within the valley floor, industrial development has created a surface that is almost entirely manmade and impervious (fig. 3; Dewitz and U.S. Geological Survey, 2021).
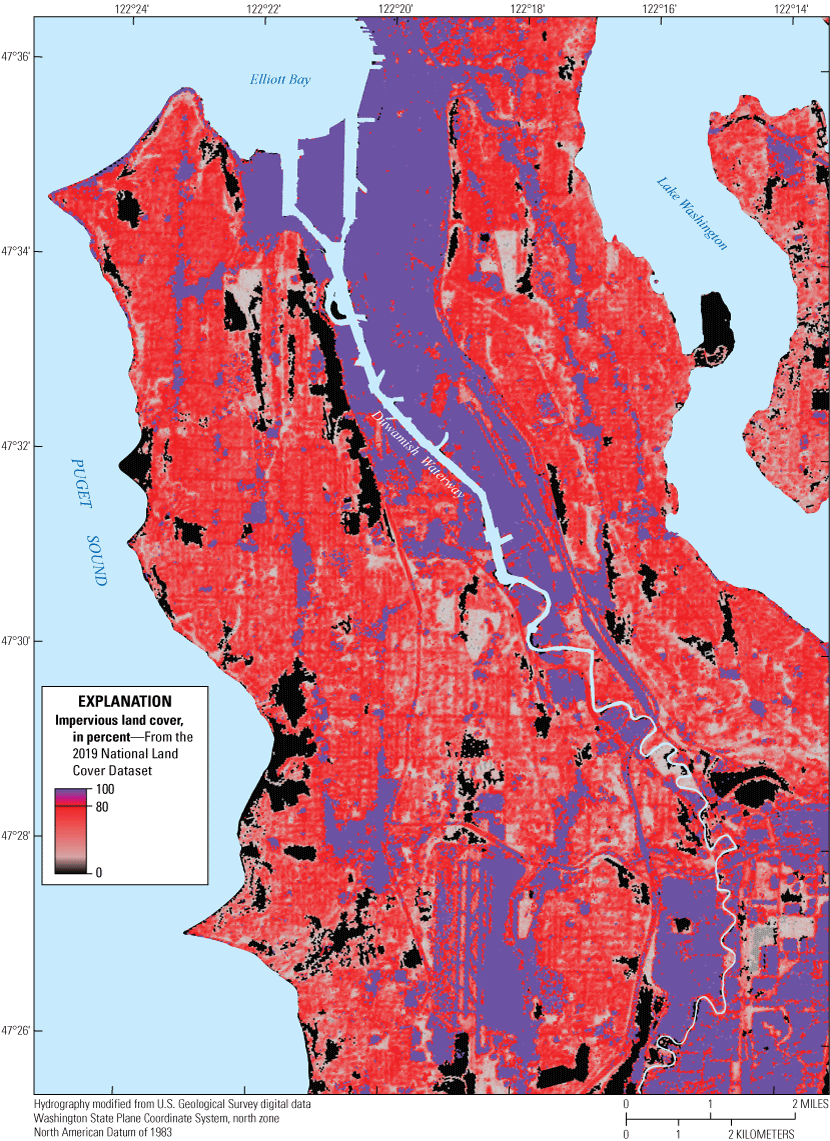
Impervious land-cover map of the area surrounding the lower Duwamish Waterway, Seattle, Washington. Land-cover data from the 2019 National Land Cover Dataset (Dewitz and U.S. Geological Survey, 2021) and has a 30-meter resolution.
Duwamish River Characteristics
Before natural and artificial alterations began in 1906, the drainage area of the Duwamish River exceeded 1,600 square miles (mi2) and included the White, Cedar, Black, and Green River Basins (Santos and Stoner, 1972). The upstream course modifications in the early 1900s included (1) the diversion of the White River to the Puyallup River to the south in 1906 to help control flooding, and (2) the lowering of Lake Washington by 9 ft in 1916 to accommodate a ship canal to Puget Sound, which resulted in the diversion of the Cedar River to Lake Washington and the reduction of the Black River to a minor stream. After upstream course modifications, the Green River became the primary source of the Duwamish River—with limited inflow from the Black River—and the drainage area for the Duwamish River was reduced to about 498 mi2 (Mastin and others, 2016; U.S. Geological Survey, 2023b). The river changes name from the Green River to the Duwamish River at about RM 12.
In addition to the upstream course modifications, the lowermost 5 mi of the Duwamish River (the lower Duwamish Waterway) were straightened in the early 1900s and have been dredged regularly since (for a comparison of the previous and current [2024] river paths, see fig. 4). The lower Duwamish Waterway is maintained as a Federal navigation channel by the U.S. Army Corps of Engineers and facilitates commerce and industry. The depth of the lower Duwamish Waterway varies from about −8 ft NAVD 88 at RM 4.8 to about −54 ft NAVD 88 near the mouth (Lower Duwamish Waterway Group, 2004). In 1961, the Howard Hanson Dam was completed on the Green River at RM 64 to regulate flows between minimum summer flows of 300 cubic feet per second (ft3/s) and maximum winter flows of 12,000 ft3/s, primarily for flood control. Mean daily streamflow at USGS streamgage 12113390, at RM 10.4, during 2014–22 was about 1,600 ft3/s (U.S. Geological Survey, 2023c).
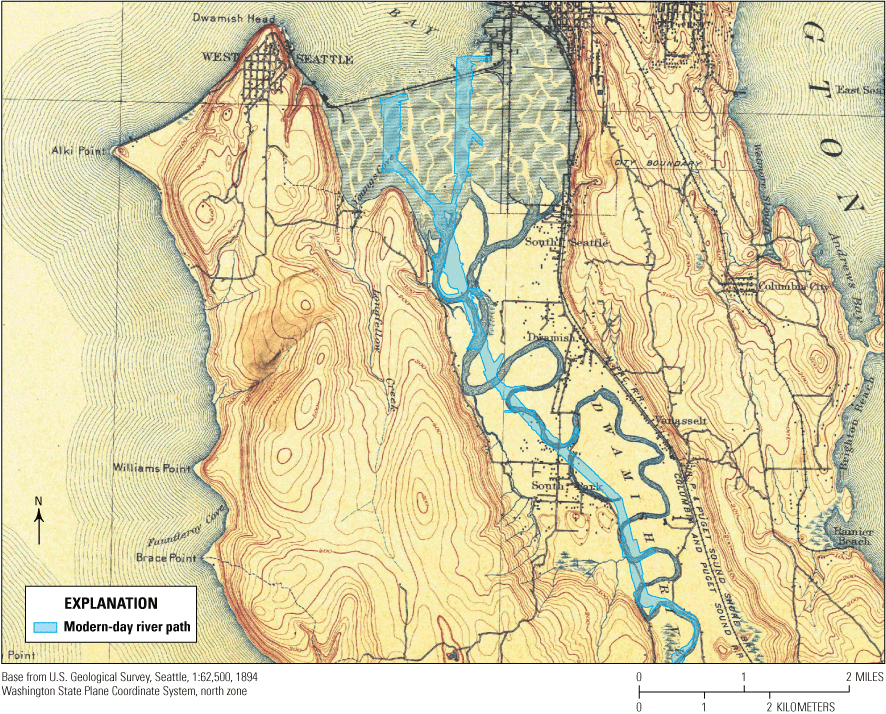
Historical topographic map showing area surrounding the lower Duwamish Waterway, Seattle, Washington (1894). The modern-day (2024) river path is overlayed. Map from U.S. Geological Survey (1894).
The Duwamish River is a stratified estuary with a large tidal range. The tides from the Pacific Ocean affect the flow and level of water in the Duwamish River and usually cause two high tides and two low tides in a day. The tidal range between mean lower low water (MLLW) and mean higher high water (MHHW)—sometimes referred to as the diurnal range—in Elliot Bay is 11.4 ft (NOAA, 2023b). The tides propagate upstream, decreasing in magnitude with distance from the river mouth; the tidal range at USGS streamgage 12113390 (RM 10.4) is about 4.2 ft (U.S. Geological Survey, 2023c).
Salt water enters the Duwamish River from its mouth at Elliot Bay and travels to a distance upstream that varies with the tides and the amount of fresh water entering upstream from the Green River (Dawson and Tilley, 1972). The intrusion of salt water occurs as a stratified wedge: denser salt water from Elliot Bay underlies the less-dense water traveling downstream from the Green River. The salinity of the lower layer is nearly identical to that of Elliot Bay, whereas the salinity of the upper layer increases downstream, starting with a value near fresh water in the Green River (Dawson and Tilley, 1972). The overriding upper layer of river water has a net downstream movement and entrains some of the salt water from the lower layer. The lower layer has a net upstream movement and contains nearly unmixed salt water. The lower saltwater layer is commonly called a salt wedge because of its shape in a longitudinal cross section; the wedge tapers upstream and the upstream-most point is known as the toe of the salt wedge (Dawson and Tilley, 1972). At discharges greater than 1,000 ft3/s, water in the Duwamish River is always fresh at least as far downstream as RM 7.8, regardless of tidal stage (Fischer and others, 1975; Conn and others, 2018). At low river discharges and high tides, the saltwater toe has been observed as far upstream as RM 10.2 (Fischer and others, 1975). Even during peak streamflows, salt water extends at least as far upstream as RM 1.9 (Stoner, 1967; Santos and Stoner, 1972; Conn and others, 2018). The position and geometry of the salt wedge fluctuate constantly, migrating up and down the Duwamish River in response to changes in the tidal stage and streamflow (Dawson and Tilley, 1972; Conn and others, 2018; McKeon and others, 2020).
The shoreline of the Duwamish River is extensively modified along the lowermost 5 mi to support the commercial uses of the waterfront (Simenstad and others, 2011). Owing to the straightening and dredging in the early 1900s, natural shoreline—that is, the pre-1900s shoreline—is generally absent in this area (Simenstad and others, 2005). Furthermore, many sections of shoreline have been armored, meaning that artificial structures were installed to prevent the natural erosion of shoreline sediments, including concrete seawalls or riprap retaining walls parallel to shore (Heerhartz and others, 2014). Morley and others (2012) mapped the lowermost 11 mi of the Duwamish River shoreline and determined that more than 66 percent of the shoreline is armored, including 86 percent of the shoreline downstream from RM 5.0.
Geology
The geology of the study area records a complex history of glaciation, faulting, unconsolidated sediment deposition, erosion, and extensive anthropogenic modifications. A summary of major geologic events and the regional stratigraphy is based on the work of Booth (1987), Galster and Laprade (1991), Booth (1994), Dragovich and others (1994), Booth and Herman (1998), Troost and others (2005), and Troost and Booth (2008).
Geologic History
The Puget Lowland—the wide low-lying area between the Cascade Range to the east and the Olympic Mountains to the west—was formed during the Tertiary as a forearc basin from the collision and subduction of the Juan de Fuca plate beneath the North American plate (Ando and Balazs, 1979). The bedrock in the Seattle area of the Puget Lowland consists of Tertiary sedimentary and volcanic rocks that underlie and fill the forearc basin. Bedrock in this region is folded into a series of west-northwest trending anticlines and synclines (Mullineaux, 1970). Bedrock characteristics in the Duwamish River valley are primarily informed by geophysical data, a few deep boreholes, sparse local exposures, and bedrock evaluations elsewhere in the Puget Lowland because Quaternary unconsolidated sediments cover much of the bedrock in the Duwamish River valley (Yount and Gower, 1991).
The Duwamish River valley is a former limb of Puget Sound, which was carved into the Puget Lowland by glacial and glaciofluvial scouring from ice sheets repeatedly advancing and retreating from British Columbia (Galster and Laprade, 1991). During each glacial sequence, the glaciers and subglacial rivers eroded and redeposited enormous volumes of sediment; these processes also can obscure evidence for previous glacial and non-glacial intervals. At least seven glacial advances have been documented in the Puget Lowland during the Quaternary (Troost and Booth, 2008). The most recent glaciation was the Vashon stade of the Fraser glaciation, which retreated from the Seattle area about 16.4 thousand years ago (ka) and, at its maximum, covered Seattle with at least 3,000 ft of ice (Armstrong and others, 1965; Booth, 1987; Porter and Swanson, 1998).
Following the last glacial retreat, the Duwamish limb of Puget Sound extended as far inland as the City of Auburn, about 20 miles south of the mouth of the current Duwamish River at Elliot Bay (Troost and Booth, 2008). About 5.7 ka, an enormous volcanic mudflow, the Osceola Mudflow, descended from the summit and northeast flank of Mount Rainier along the White River valley, spilling into the Green and Puyallup River drainages (Dragovich and others, 1994). The voluminous deposits from the Osceola Mudflow were then re-eroded in the upper valleys and deposited by rivers farther downstream, leading to rapid progradation of the deltaic sediments. Sediment deposition in the ancestral White River—what is now the Green River valley—gradually moved the shoreline of the Duwamish limb of Puget Sound northward to its current location at Elliot Bay (Dragovich and others, 1994). Sediment deposition after the Osceola Mudflow almost completely buried the previous form of the valley with fine silts, sands, and gravels associated with riverine and floodplain deposits.
Several active or recently active fault zones underlie the Puget Lowland (Troost and Booth, 2008). The primary source of the tectonic stress is the ongoing subduction of the Juan de Fuca plate beneath the North America plate (Galster and Laprade, 1991; Blakely and others, 2002). The east-west trending Seattle Fault Zone traverses the Duwamish River valley over RMs 0–4.5 and separates the Seattle basin (north) from the Seattle uplift (south). Owing to a large earthquake about 1.0 ka, land north of the fault subsided by as much as 3 ft and land south of the fault was uplifted by as much as 20 ft (Bucknam and others, 1992).
In the most recent geologic phase for the Duwamish River valley, the tideflats and floodplain were filled and the river was straightened and dredged in the early 1900s to enable improved waterway navigation for commerce. The sediments dredged from the new channel, along with additional sand and other materials sourced elsewhere, were used to fill the abandoned river channel and lowlands above flood levels (Carlson, 1950). Subsequent industrial development along the river corridor resulted in the placement of mixed fill materials. As a result, the surficial and shallow geology in the river valley is a heterogeneous mixture of unmodified alluvium, reworked material from dredging, and non-native sands; in many places, these materials are difficult to distinguish (Troost and others, 2005; Troost and Booth, 2008).
Regional Stratigraphy
The geologic history of the area is reflected in the assorted deposits and landforms in the valley and adjacent uplands. The regional stratigraphic units in Duwamish River valley are described in the sections that follow, from oldest to youngest, and are grouped into three primary geologic assemblages: bedrock; glacial and non-glacial unconsolidated sediments in the uplands; and alluvial deposits and artificial fill in the valley. A simplified version of the surficial geology from Troost and others (2005) is shown in figure 5 (for more details, see the original publication).
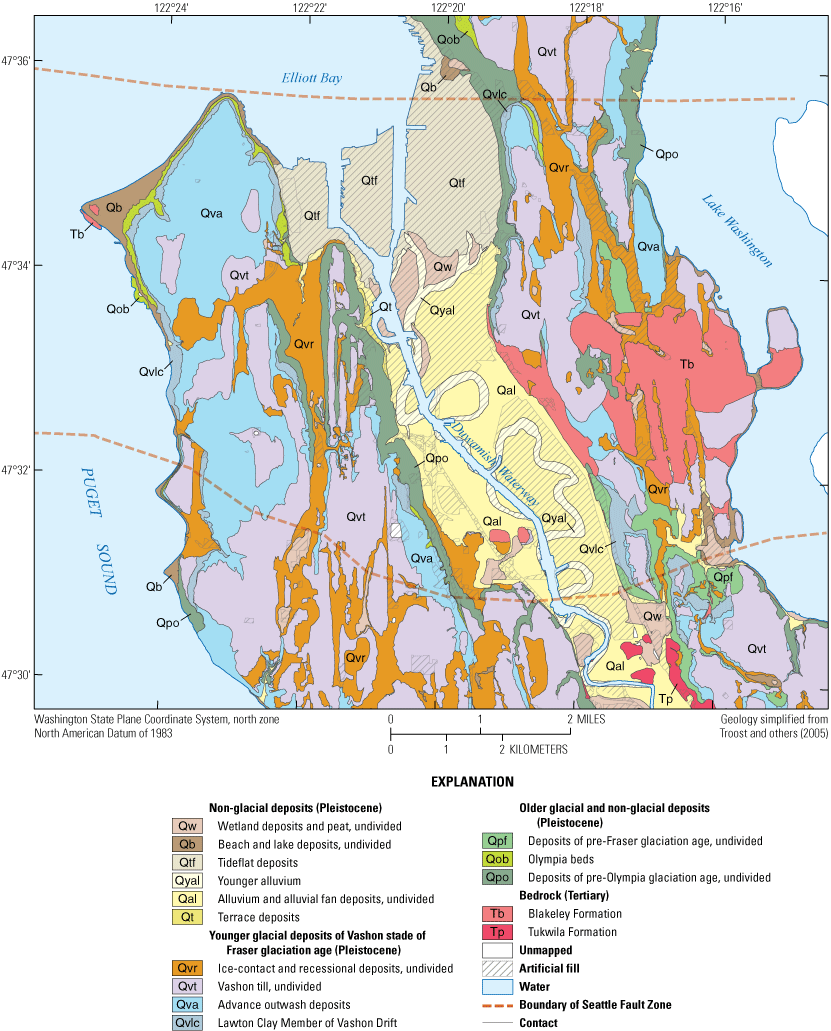
Surficial geologic map showing area surrounding the lower Duwamish Waterway, Seattle, Washington. Geology is modified from Troost and others (2005); undivided units are combined from geology of Troost and others (2005) to simplify map.
Bedrock
Exposed bedrock in the Duwamish River area consists of the Blakeley Formation (Weaver, 1916) and the Tukwila Formation of the Puget Group (Vine, 1962). The oldest unit is the Tukwila Formation, a largely sedimentary, middle- to late-Eocene deposit that accumulated near a volcanic center (Troost and others, 2005; Troost and Booth, 2008). Exposures of the Tukwila Formation in the Duwamish River region are limited to a small area near RM 6 (Troost and others, 2005). The most extensive bedrock unit is the Blakeley Formation from the Eocene and Oligocene epochs, which has large exposures to the east of the Duwamish River from RMs 1 to 4. The Blakeley Formation consists of marine sedimentary and volcaniclastic rocks deposited in a shallow marine sandy shelf environment (Troost and Booth, 2008; Haugerud and others, 2017). The Tukwila Formation and Blakeley Formation have estimated maximum thicknesses greater than 3,000 ft, based on extensive exposures to the east (Troost and others, 2005; Troost and Booth, 2008). Additional bedrock units in Seattle include the Renton Formation of the Puget Group and an unnamed assemblage of intrusive andesite and basalt, all of which lie stratigraphically between the Tukwila Formation and Blakely Formation (Troost and others, 2005); however, these other bedrock units have limited exposures in the study area and will not be further characterized here. The depth to bedrock in the Duwamish River area ranges from 0 to greater than 2,000 ft and generally increases with distance downstream and distance away from the Duwamish River (Yount and others, 1985; Jones, 1996; Eungard, 2014).
Upland Deposits
Overlying the Tertiary bedrock are the discontinuous and complex remains of at least seven Pleistocene glaciations and the corresponding non-glacial periods (Booth and others, 2003; Troost, 2016). Deposits predating the Vashon stade of the Fraser glaciation—the most recent glaciation, which reached its maximum extent about 16.9 ka (Thorson, 1980; Porter and Swanson, 1998)—are grouped into deposits of pre-Olympia glaciation age, deposits of Olympia glaciation age, and deposits of pre-Fraser glaciation age. These deposits are less well known than the deposits of Vashon-stade age because each glacial advance would have partially eroded and buried the previous deposits. The older sediments are very compact, owing to the weight of subsequent glaciations, and are commonly cemented by oxidation (Booth and Herman, 1998). Confident identification of each glacial and non-glacial depositional sequence often requires laboratory analyses and age determinations (Troost, 1999).
The deposits of pre-Olympia glaciation age in the Duwamish River area include an assortment of named and unnamed glacial and non-glacial deposits dating from the oldest Pleistocene glacial interval (200 ka) to the onset of the Olympia non-glacial interval (70 ka; Armstrong and others, 1965; Minard and Booth, 1988). This sequence of deposits records interfingering glacial advances from the north and volcanic eruptions from Mount Rainier to the southeast, both superimposed on the steady westward transport of sediment from the Cascade Range by rivers (Booth and Herman, 1998). Although age dating has allowed some deposits of pre-Olympia glaciation age to be named and reliably identified (for example, the informal Hamm Creek formation of Troost and others [2005] and Possession Drift), each of the deposits of pre-Olympia glaciation age separated by Troost and others (2005) have limited exposures and are grouped together for the purposes of this study. The deposits of pre-Olympia glaciation age contain 3–250-ft thick layers of mixed characteristics, including silt and clay, coarse glacial till, peat, and tephra (Troost and others, 2005).
The deposits of Olympia glaciation age are defined as the non-glacial strata deposited during the interglacial period immediately preceding the last major glaciation: the Vashon stade of the Fraser glaciation (Armstrong and others, 1965; Minard and Booth, 1988; Troost and Booth, 2008). The Olympia beds accumulated during 70–18 ka and have an estimated thickness of as much as about 80 ft (Troost and others, 2005). They represent a variety of depositional environments and are characterized by sand, silt, gravel, and peat, along with a relatively high amount of organic matter.
The deposits of pre-Fraser glaciation age are a map unit used “where no age control is available other than that the deposits are older than Vashon Drift, and that the deposits’ origins are indistinguishable between glacial and nonglacial” (Troost and Booth, 2008, p. 25). The deposits of pre-Fraser glaciation age can be further subdivided based on grain size and include interbedded sand, gravel, silt, and diamicts (Troost and others, 2005). The individual layers have estimated maximum thicknesses ranging from 20 to 80 ft and exposures in the study area are limited to the east of the Duwamish River.
Deposits of Vashon stade of the Fraser glaciation, in contrast to the underlying sediments, are abundant at or near the land surface and are relatively easily recognized. The oldest member of the Vashon stade age deposits is the Lawton Clay Member of Vashon Drift (Mullineaux and others, 1965), which marks the transition from a non-glacial to glacial depositional environment and is characterized by clay, silt, and dropstones deposited in a pro-glacial lake (Troost and Booth, 2008). As the Puget lobe of the Cordilleran ice sheet progressed south, a sequence of advance outwash was deposited that consists of well-sorted sand and gravel, with occasional lenses of silt (Troost and others, 2005). Overlying the advance outwash is a dense, thin layer (3–30 ft) of relatively impervious till composed of poorly sorted silt, sand, and gravel; the till is present on the surface of about one-fourth of the study area (Troost and others, 2005; Haugerud and others, 2017). As the Puget lobe melted and receded, it deposited ice-contact drift—consisting of intercalated till and outwash—and recessional outwash. The recessional outwash can be further divided into coarse-grained deposits, with sparse outcrops to the east and west of the valley, and more common fine-grained lacustrine deposits (Troost and others, 2005).
Alluvial Deposits and Artificial Fill
After deglaciation of the Seattle area, unconsolidated sediment deposition—including terrace deposits, beach and lake deposits, alluvium, tideflat deposits, wetland deposits, peat, and artificial fill—covered the trough of the Duwamish River valley and the nearby coastlines. The most extensive deposits are tideflat deposits and alluvium, which account for about 80 percent of the exposed Holocene material (Troost and others, 2005). The tideflat deposits are limited to the land adjacent to and north of the mouth of the Duwamish River and consist of silt, sand, organic sediment, and detritus; the deposits have an estimated maximum thickness of about 200 ft at the north end of Harbor Island and thin to the south.
Alluvium consisting of sand, silt, gravel, and cobbles covers most of the Duwamish River valley. The thickness of the alluvial deposits varies from a few 10s of feet to 100 ft in river and stream valleys, and generally thins to the south. The boundary of the alluvial deposits is either bedrock, where present, or dense, glacially overridden sediments (Booth and Herman, 1998). Estuarine and marine deposits form the base of the alluvial sequence and grade upward into more complexly interbedded fluvial sand, silt, and gravel (Galster and Laprade, 1991). The surficial geologic map of Troost and others (2005) identifies the sediment used to fill the historical Duwamish River channels as younger alluvium (unit Qyal, fig. 5); this is distinguished from the younger alluvium stratigraphic unit described by Booth and Herman (1998, pls. 3–5), which generally refers to all alluvium deposited “by the modern Duwamish River while flowing at an altitude substantially equivalent to that seen today.” The younger alluvium unit of Booth and Herman (1998) covers most of the Duwamish River valley and is within a few feet above or below 0 ft NAVD 88 (which is near modern [2024] sea level), whereas the older alluvium unit of Booth and Herman (1998) refers to sediment transported by the Duwamish River while the valley floor was still between tens to hundreds of feet underwater—that is, the older alluvium unit is entirely covered by younger alluvium. For the remainder of this report, the division of younger and older alluvium by Booth and Herman (1998) is used, unless otherwise indicated, because it is useful for describing the varying hydrogeologic characteristics of the alluvial material in the subsurface. The younger alluvium is characterized by wood and organics in a silt and sand matrix and is generally limited to the shallowest 15–20 ft of the subsurface (Booth and Herman, 1998). The younger alluvium grades downward into the older alluvium, which consists of a coarser, predominantly sandy alluvium with smaller quantities of silt and less organics. The thickness of the older alluvium ranges from 10 to 30 ft in the upper valley (about RMs 5–7) to 150–200 ft (about RMs 0–1.5; Booth and Herman, 1998). Artificial fill—consisting of heterogeneous gravel, sand, silt, concrete, and garbage—discontinuously covers much of the valley floor and ranges in thickness from a few feet to a few tens of feet (Troost and others, 2005).
Data and Methods
Available information was compiled and new data were collected to describe the regional groundwater system and site-specific groundwater and surface-water interactions. Owing to the EPA Superfund status of the lower Duwamish Waterway, many site-specific hydrogeologic investigations have been completed in the area by various consultants and organizations, in addition to a smaller number of regional studies. A bathymetric survey was completed to understand the interface of groundwater and surface-water interactions at a Nearshore Site where new groundwater data were being collected. Groundwater-level data (hereinafter, water-level data) from past studies were combined with new water-level data to generate potentiometric maps and evaluate the tidal influence on water levels. Water levels in this study are referenced to the NAVD 88.
Data Compilation
For this study, data were compiled from (1) site-specific remedial investigations, (2) regional (Duwamish River valley) investigations, and (3) online databases. Site-specific remedial-investigation reports—related to contamination of groundwater, sediments, and the Duwamish River—are abundant along the lower Duwamish Waterway and were primarily sourced from the Washington State Department of Ecology’s online database for cleanup sites (Washington State Department of Ecology, 2023f). Regional groundwater studies in the Duwamish River valley with useful data and hydrologic information include the conceptual model from Booth and Herman (1998) and the remedial-investigation report from the Lower Duwamish Waterway Group (2010). These reports contain water-level and water-quality data and additional hydrogeologic information (for example, lithologic descriptions and hydraulic testing from boreholes) to help characterize the study area. In some reports, the data were presented in a tabular format that allowed for easy extraction and reuse. In other reports, the data of interest were only presented in maps or figures and required more laborious extraction and, for geospatial data extracted from maps, georeferencing. Online databases containing water-level and water-quality data used in this study include (1) the Washington State Department of Ecology’s Environmental Information Management System (Washington State Department of Ecology, 2023d) database, (2) the USGS National Water Information System (NWIS; U.S. Geological Survey, 2023c) database, and (3) the Washington State Department of Natural Resources’ Subsurface Geology database (Washington State Department of Natural Resources, 2023). Data compiled from these resources include water-level and specific-conductance data and lithologic and well-construction data from well logs. Only groundwater wells with reliable altitudes and well-construction information (for example, wells where the total depth and depth of the open interval[s] are known) were used.
Data Collection at a Nearshore Site
A nearshore site (hereinafter referred to as “Nearshore Site” to distinguish the particular site from general discussions of nearshore areas) along the western shoreline of the Duwamish River from RMs 1.4 to 1.5 was selected for focused groundwater data collection by USGS (fig. 6). A bathymetric survey was conducted in the embayment to better understand the interface for groundwater and surface-water interactions at the site. Data loggers were deployed in seven groundwater wells and one stilling well in the Duwamish River (table 1). The data loggers measured specific conductance, temperature, and depth at 15-minute intervals for a period of about 2 years. The groundwater wells had depths ranging from 10.0 to 24.5 ft. The suffix of each well’s common name corresponds to either shallow (“S”) or deep (“D”), which refers to the relative depth of the open interval in the well. Two pairs of wells (MW-14S and MW-14D; and MW-45S and MW-45D) were co-located and were open to different depths.
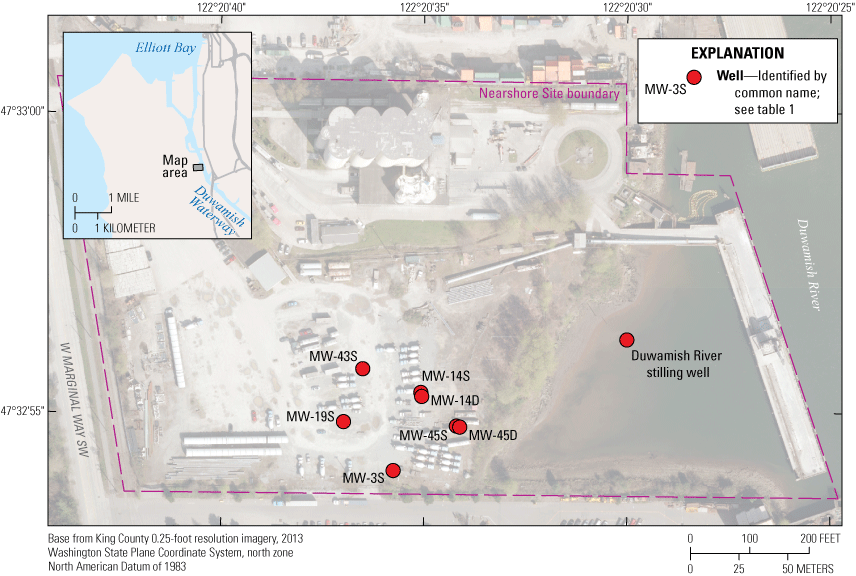
U.S. Geological Survey monitoring locations at the selected Nearshore Site along the lower Duwamish Waterway, Seattle, Washington. Monitoring locations are identified by their common name (table 1).
Table 1.
Characteristics of data-collection sites in the Duwamish River valley, Washington (ERM-West, Inc., 2020; U.S. Geological Survey, 2023c).[Abbreviations: USGS, U.S. Geological Survey; MW, monitoring well; MP, measuring point; NAVD 88, North American Vertical Datum of 1988; WA, Washington State]
Embayment Bathymetry Survey
A bathymetric survey of the embayment between the shoreline near the selected Nearshore Site and the main channel of the Duwamish River was conducted on January 19, 2021. The survey used a Global Positioning System-equipped acoustic Doppler current profiler (ADCP) that was towed behind a watercraft in transects parallel to the shoreline and across the open embayment. The survey occurred during a 2-hour interval straddling high tide, and the water level only fluctuated by about 0.46 ft during the survey. The USGS velocity mapping toolbox (Parsons and others, 2012) was used to process the ADCP data.
Water-Level, Temperature, and Specific-Conductance Data Collection
Each of the seven groundwater wells was equipped with a data logger, which was programmed to continuously record absolute pressure, temperature, and specific conductance at 15-minute intervals. Data loggers were suspended in the wells from a fixed point near the top of the well casing. Absolute pressures recorded by the non-vented pressure transducers were converted to submergence depths by subtracting the concurrent barometric pressure recorded by an on-site barometer, which resulted in water-submergence pressures. The water-submergence pressures were then multiplied by a constant value of 2.307 to convert to water-submergence depths—1 pound per square inch is about 2.307 ft of water depth. (The non-vented pressure transducers have an accuracy of about 0.03 ft [In Situ Inc., 2023].) The continuous submergence-depth data were converted to water levels by applying offsets to about match the discrete water-level measurements collected during site visits by applying offset-correction values to the data recorded immediately before and after each site visit. The offset-correction values were prorated linearly between consecutive site visits. To determine the offset-correction values, the continuous water-level records were visually extrapolated to the discrete water-level measurement nearest in time. Such extrapolation was necessary because the water levels can change by a few hundredths of a foot during the time between the discrete water-level measurement and the first recording by a transducer. Most often, the extrapolation spanned 10 minutes or less. The water-level data, although reported to hundredths of a foot, have several components that could affect their accuracy. The continuous water-level data are only as accurate as the discrete water-level data because the beginning and ending of each individual water-level record collected between site visits are referenced to manual depth-to-water measurements. Factors that could adversely affect the accuracy of the discrete water-level data include but are not limited to (1) the accuracy of the water-level tape used to collect the measurement, (2) the accuracy of the surveyed measuring-point altitude, and (3) inconsistent measurement practices.
Water-temperature data, in degrees Celsius (°C), were recorded by each data logger and had an accuracy of about 0.1 °C (In Situ Inc., 2023). Specific-conductance data were recorded in microsiemens per centimeter (µS/cm) and had an accuracy that varied from about 1 to 250 µS/cm, depending on the value of the reading—the higher readings were less accurate (In Situ Inc., 2023). Specific conductance is an easily measured proxy for salinity that compensates for variations in fluid temperature, meaning that the measured value is corrected to what it would be at 25 °C. Data loggers were operated following standard USGS methods (Wagner and others, 2006; Cunningham and Schalk, 2011), which include pre- and post-deployment calibration checks and regular site visits. The data-review process included applying corrections or deletions of data owing to calibration drift or sensor fouling (Wagner and others, 2006; Cunningham and Schalk, 2011). For example, data are missing from four sites from August 19, 2021, to February 2, 2022, during periods of equipment malfunction. Approved data are publicly available in the USGS NWIS database (U.S. Geological Survey, 2023c).
Potentiometric-Map Development
Potentiometric-surface maps were created with available hydraulic-head data to evaluate hydraulic gradients and groundwater-flow directions in the study area. The potentiometric surface represents the level to which water will rise in a well that is exclusively screened within a given aquifer; that is, the potentiometric surface is often different for aquifers at different depths. In an unconfined aquifer, the hydraulic head is equal to the water table; in a confined aquifer, the hydraulic head is equal to the level to which water will rise if the aquifer was pierced by a well that is only screened within the confined aquifer. For the remainder of this report, the term “water level” is used in place of hydraulic head, independent of the unconfined or confined condition of the aquifer(s) being discussed. Outliers in the water-level data were uncommon; however, if a reasonable explanation could not be found, outliers were removed from further analysis. Potential sources of error for the outliers include errors in data entry or the incorrect use of a vertical datum.
A regional water-table map (a type of potentiometric-surface map) was developed. To select wells representing the water table—the shallowest, unconfined aquifer for an area—a criterion was established such that only wells where the top of the screened interval was less than 20 ft below the ground surface were used; 20 ft is the approximate depth of the low-permeability layer in the river valley that sometimes separates the water table from a deeper semi-confined or confined aquifer. This criterion was not applied outside the river valley in areas where no other water-level data were available. For wells with continuous water-level data or multiple discrete water-level measurements, a mean water level was computed. At wells near the Duwamish River where tidal variability may affect the water level in the well, the water levels used to compute the mean water level were reviewed to ensure that the computed mean water level was representative. Available data were used to generate a potentiometric surface with the Topo to Raster tool in ArcGIS Pro. The final regional contour map shows solid and dashed lines to distinguish between areas with more and less confidence in the contour value, respectively. The dashed contours indicating the approximate location of a contour line represent areas farther than 500 ft from a well with a water-level value used to generate the potentiometric surface.
Additional potentiometric-surface maps were developed for areas surrounding the selected Nearshore Site (fig. 6). Potentiometric surfaces for the shallow and deep aquifers—distinguished using the 20-ft top-of-screen-depth criterion—for the site were created that represent aquifer conditions at low, mean, and high tides. For a well to be included in the potentiometric-map development for low- and high-tide conditions, it must have had either (1) continuous water-level data, from which the low- or high-tide water levels were identified and averaged, or (2) sufficient discrete water-level measurements with the tidal status identified by the original source, which were then averaged. For wells outside the zone of tidal influence and with continuous water-level data, the times of the low- and high-tide water levels determined for nearby tidally affected wells—from the same study or source and, thus, with concurrent time periods—were used to select concurrent water levels from the non-tidally affected wells. If both continuous and discrete water-level data were available, the continuous water-level data were given preference, all other factors being equal, because of the higher confidence in the tidal status of the consequent water level. Owing to concerns about the accuracy, or absence, of times recorded for discrete water-level measurements at some sites, the tidal stage recorded in Elliot Bay, Seattle, was not used to estimate the tidal status for discrete water-level measurements where the tidal status was not explicitly identified by the original source. For the mean-tide potentiometric surface, wells with only discrete water-level measurements were reviewed to ensure that the well was outside the zone of the tidal influence or that the discrete water levels were representative of mean tide. The Series (1991) method for determining the mean water level at tidally affected wells was used for wells with continuous water-level data.
The water-level data used to generate the potentiometric surfaces are from different sources and time periods (in the case of the regional water-table map, some data were collected before 1998 [as shown in appendix B of Booth and Herman, 1998]); consequently, the resulting potentiometric surfaces only represent the general groundwater-flow directions and are not true synoptic water-level surveys. For the potentiometric surfaces created for the Nearshore Site, efforts were made to ensure that data were comparable and represent similar tidal and seasonal signals; this is especially important for the shallow wells at the Nearshore Site, where the seasonal signal is dominant.
Evaluation of Site-Specific Characteristics at a Nearshore Site
Groundwater data were collected at a Nearshore Site along the western shoreline of the Duwamish River from RMs 1.4 to 1.5 to help characterize groundwater and surface-water interactions in the lower Duwamish Waterway. The site officially spans about 18 acres, including the 4-acre embayment. The Nearshore Site was selected because of its existing monitoring-well network, known contamination, and wade-able access to the intertidal zone and nearshore shallow water. The site has hosted a variety of industrial businesses since the middle of the 20th century, including chemical manufacturing, activated-charcoal manufacturing, and cement storage and distribution. Contamination at the site is primarily related to the historical uses and manufacturing of pentachlorophenol, glues, and resins (Washington State Department of Ecology, 2023b). The site is one of several sites that will be cleaned up as part of Ecology’s Source Control Strategy (Washington State Department of Ecology, 2023c) and is undergoing a remedial investigation and feasibility study (RI/FS). The draft RI/FS was published in 2020 (ERM-West, Inc., 2020) on Ecology’s site-cleanup website (Washington State Department of Ecology, 2023b). Although the report is only available in draft form, it contains continuous water-level data from a tidal study conducted in 2013 (ERM-West, Inc., 2020, app. J) and discrete water-level data from 2009 to 2018 (ERM-West, Inc., 2020, table 2-1) that will be combined with newly collected water-level data.
The draft RI/FS identified seven distinct lithologic units at the site, from deepest to shallowest: lower sand; silt aquitard; native soil; dredge fill; bank fill; shallow fill (including gravel fill); and surface coverings (ERM-West, Inc., 2020). The lower sand unit is saturated and laterally continuous at the site and represents the older alluvial unit of Booth and Herman (1998); the depth to the top of the unit varies from about 15 to 20 ft below ground surface. A clayey silt aquitard also containing organic material overlies the lower sand unit and is interpreted as the intertidal-wetland surface prior to the anthropogenic placement of fill. The silt aquitard is continuous across the site and has a thickness ranging from 3.5 to 12 ft (ERM-West, Inc., 2020). Native soils interfinger and overlie the silt aquitard on the west side of the site. The remaining sequence of fill materials represents intermittent human activities spanning the 20th century and is characterized by a heterogeneous mixture of sand, silt, organics, gravel, and construction debris. Much of the site’s surface has been developed with impervious infrastructure (for example, buildings and roads); however, the southern part of the site remains mostly unpaved and is covered with gravel.
Two saturated zones have been identified in the subsurface at the site (ERM-West, Inc., 2020). The deep (or lower) zone is a confined aquifer in the lower-sand unit that lies beneath the silt aquitard. Sediment in the deep zone is finer than in the shallow zone, and, owing to the weight of the overlying ice sheets during recent glaciations, is also dense and relatively compact. The shallow (or upper) zone is an unconfined aquifer overlying the silt aquitard at depths from about 4 to 13 ft below ground surface. The shallow aquifer occurs within the shallow fill and dredge fill lithologic units.
The historical path of the Duwamish River crossed the easternmost 100–150 ft of the current land surface at the site and was subsequently filled in the early 1900s. Although classified as riprap and bulkhead in the lower Duwamish Waterway-shoreline survey conducted by Morley and others (2012), the shoreline at the site is characterized by bank-fill sediments interspersed among riprap and partially buried bulkhead; that is, the shoreline is discontinuously armored and the armoring is unlikely to represent an impermeable barrier to groundwater flow.
Water Levels
Water-level data recorded at seven groundwater wells and a stilling well in the Duwamish River during 2020–22 revealed distinct hydrogeologic regimes (fig. 7). Water levels in the shallow wells were 3–8 ft higher than water levels in the deep wells, providing further evidence for the low permeability layer between the shallow and deep aquifers in this area. Data from the shallow wells had a pronounced seasonal variability, with high water levels in the winter and low water levels in the summer; the deep wells showed far less seasonal variability, with slight increases in the winter and a near constant water level from spring to autumn. For the two sets of paired shallow/deep wells (MW-14S and MW-14D; MW-45S and MW-45D), the vertical hydraulic gradient ranged from about −0.3 to −0.4 foot per foot (ft/ft), indicating flow in the downward direction (that is, from the shallow zone to the deeper zone). The downward vertical gradient was greatest in winter when water levels in the shallow wells rose owing to increased rainfall. In addition to the seasonal increase in water levels, water levels in the shallow and deep wells showed a similar short-term increase following heavy precipitation (fig. 8); select water-level data in fig. 8A were smoothed using a locally weighted scatterplot smoothing to highlight the response to precipitation.
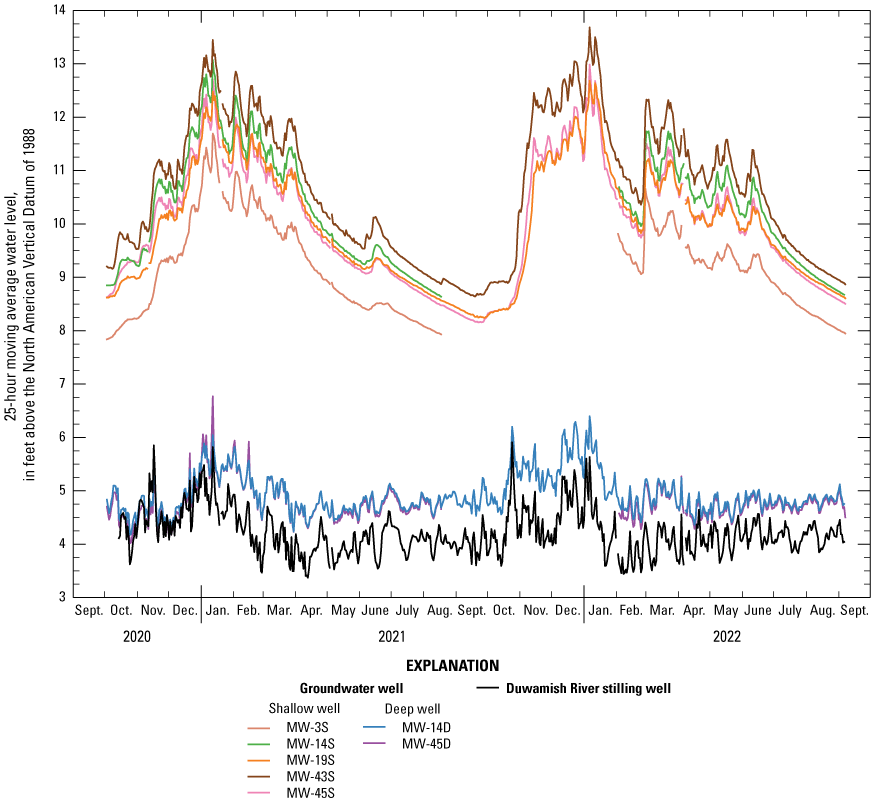
Graph showing 25-hour moving average water levels recorded at monitoring locations at the selected Nearshore Site along the lower Duwamish Waterway, Seattle, Washington, 2020–22. The 25-hour moving average was chosen because it approximates the period of the tidal cycle.
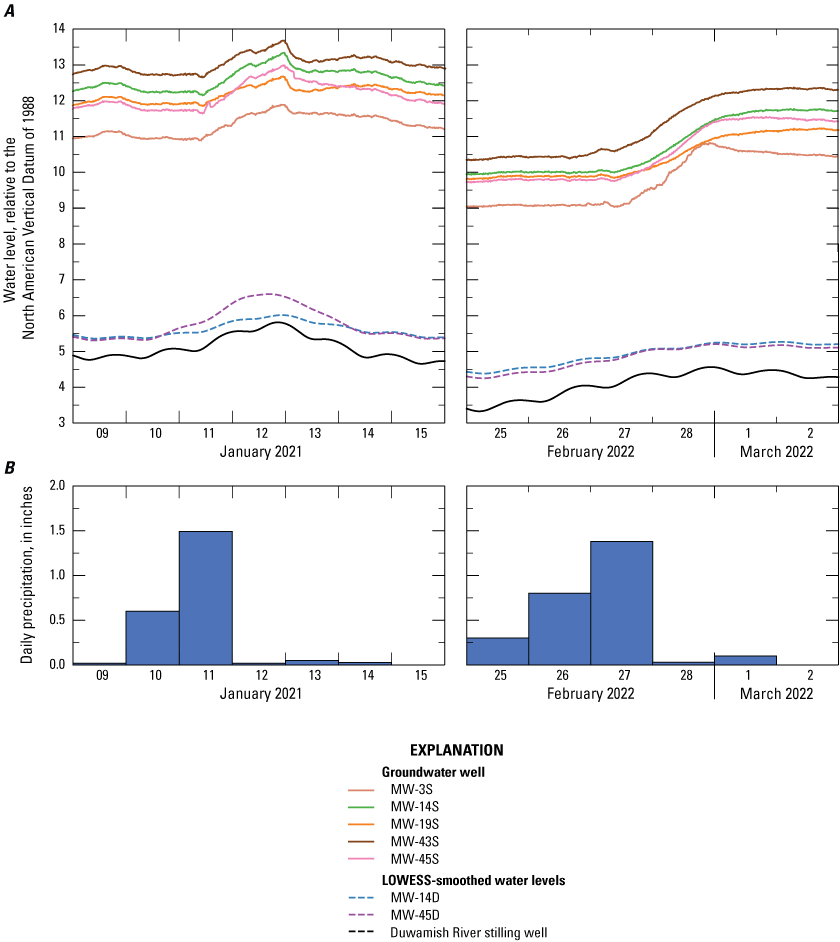
(A) Water levels in shallow monitoring wells and locally weighted scatterplot smoothed (LOWESS) water levels in deep monitoring wells and the Duwamish River stilling well, and (B) the daily precipitation recorded at Boeing Field Airport, Seattle, Washington, January 9–15, 2021 (graphs on left), and February 25–March 2, 2022 (graphs on right). Precipitation data from National Oceanic and Atmospheric Administration (2023a).
The deep wells have a strong hydraulic connection to the Duwamish River, as evidenced with the synchronous tidal variability in groundwater levels and specific conductance (fig. 9). A tidal response is present in the water-level records of the shallow wells; however, the tidal variation is only about 0.01–0.03 ft and is barely perceptible. The time lag of tidal peaks and troughs for MW-14D and MW-45D were about 70 and 60 minutes, respectively. The tidal stage ratio—the ratio of the groundwater-level change to the river-stage change—for MW-14D and MW-45D was about 0.34 and 0.36, respectively.
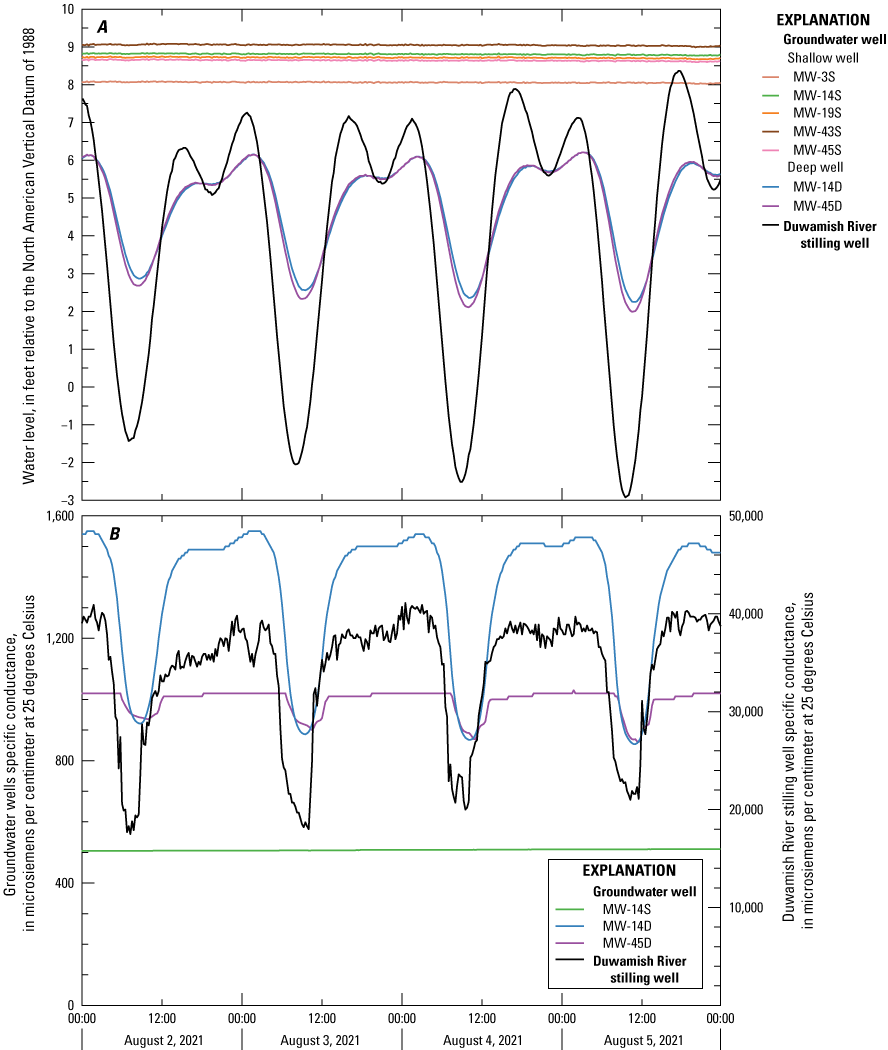
(A) Water level, and (B) specific conductance recorded at monitoring locations at the selected Nearshore Site along the lower Duwamish Waterway, Seattle, Washington, August 2–5, 2021.
Water levels in the tidally affected deep wells are slow to decline after the tidal peak. That is, for a given river stage, the water level will be greater in a nearby deep well if the river stage is on the falling limb of a tidal cycle than the rising limb of a tidal cycle (fig. 10). The water-level hysteresis shown in figure 10 is indicative of bank storage and the delayed release of water from near-river sediments and is also a consequence of the time lag in response to tidal forcing. The hysteresis pattern observed in the deep wells at the Nearshore Site is also likely present in shallow wells elsewhere along the lower Duwamish Waterway without a prominent confining unit, and, thus, more of a tidal influence in the shallow wells.
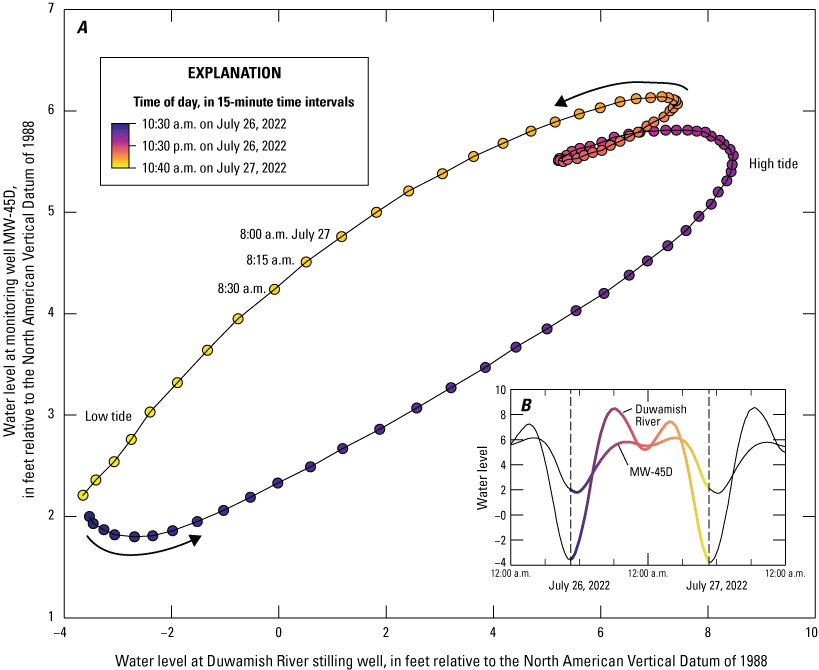
(A) The relation between water levels in monitoring well MW-45D and the Duwamish River stilling well during one tidal cycle, and (B) the same water levels relative to time, along the lower Duwamish Waterway, Seattle, Washington, February 26–28, 2022.
Water-level data from the 2020 RI/FS report (ERM-West, Inc., 2020) were used to evaluate the tidal changes in flow direction and hydraulic gradient in the nearshore areas. The 10-minute water-level data were resampled to hourly water levels, and the direction and slope of the hydraulic gradient was computed for pairs of water levels and the nearest shoreline point corresponding to the concurrent river stage. For each time step, the water level in the river was used to determine the point on the shoreline corresponding to the given river stage that minimized the squared distance between both wells. These three points (the two wells and the nearest shoreline point for the given river stage) were used to generate a plane that intersects the water-level altitudes associated with each point. The shaded shoreline circles in figure 11 represent the average location of the shoreline point that was used to compute the slope and gradient for each time step. These data reveal the reversal of flow directions with changes in tidal stage and the localized differences in flow directions (fig. 11).
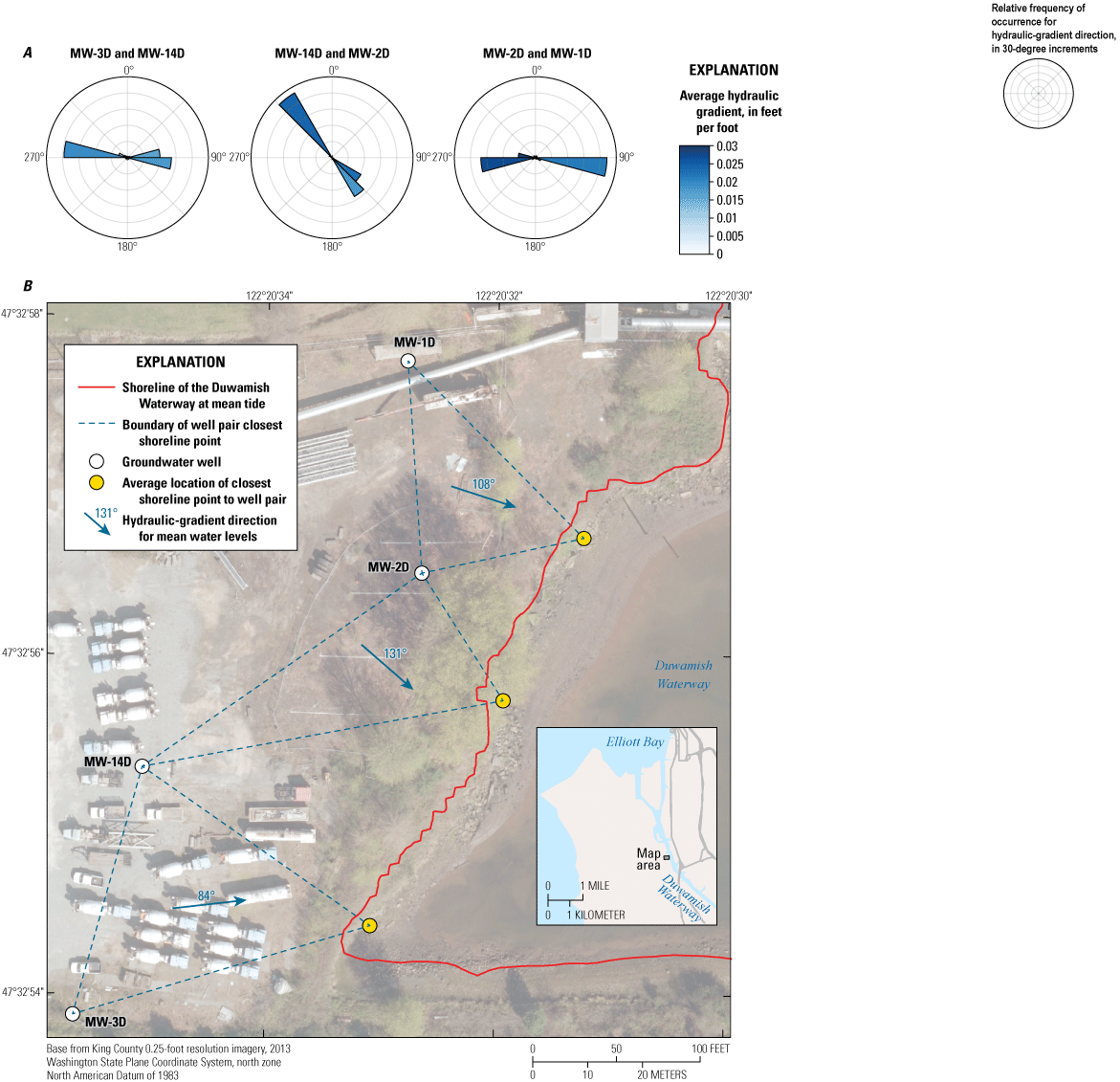
Polar bar charts showing the relative frequency of occurrence for hydraulic-gradient directions using water levels from two groundwater monitoring wells (A) and map showing the concurrent stage of the Duwamish River using 10-minute data (B), along the lower Duwamish Waterway, Seattle, Washington, July 18–27, 2013. The water-level data are from ERM-West, Inc. (2020, app. J).
The potentiometric maps developed for the Nearshore Site and surrounding areas indicate large differences in groundwater-flow directions for the shallow and deep aquifers at low and high tides (fig. 12). For the shallow aquifer, flow is toward the lower Duwamish Waterway near the Nearshore Site, regardless of the tidal condition (fig. 12A–C). For the deep aquifer, a potentiometric trough forms parallel to the shoreline during high tide, indicating that groundwater flow converges from the uplands to the west and the Duwamish River to the east (fig. 12F). Potentiometric-surface-difference maps—created by subtracting the potentiometric surface of the deep aquifer from that of the shallow aquifer—suggest large vertical downward gradients at the Nearshore Site at each tidal condition (fig. 13). The downward gradients are greatest during low tide because the potentiometric surface of the deeper aquifer follows the tidal cycle of the river whereas the potentiometric surface of the shallow aquifer is relatively unaffected.
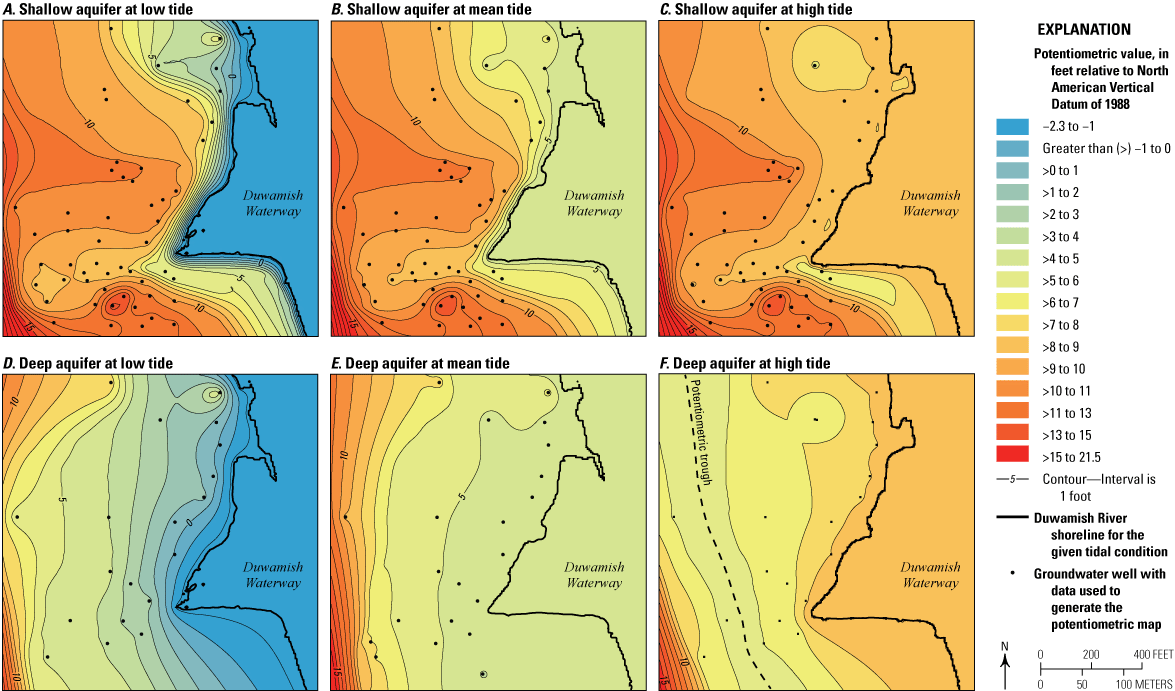
Potentiometric-surface maps for the shallow and deep aquifers during low, mean, and high tide at the selected Nearshore Site along the lower Duwamish Waterway, Seattle, Washington. (A) shallow aquifer at low tide, (B) shallow aquifer at mean tide, (C) shallow aquifer at high tide, (D) deep aquifer at low tide, (E) deep aquifer at mean tide, and (F) deep aquifer at high tide.
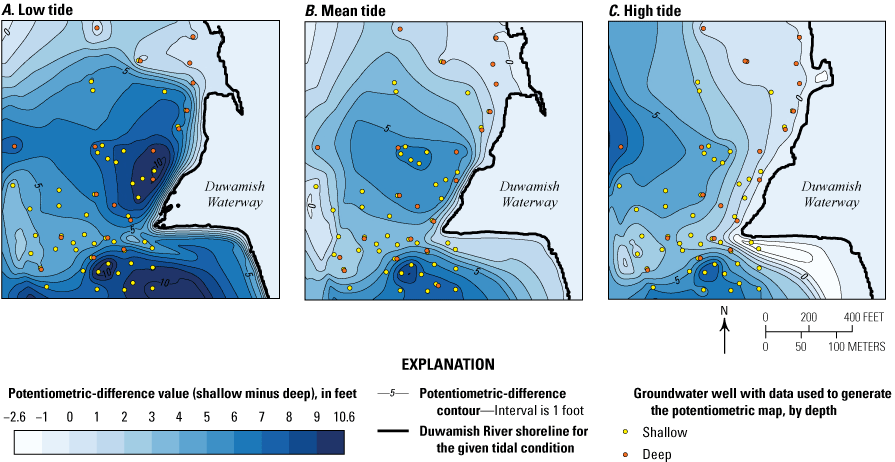
Potentiometric-surface-difference maps during (A) low, (B) mean, and (C) high tide at the selected Nearshore Site along the lower Duwamish Waterway, Seattle, Washington.
Potentiometric-surface maps were combined with topobathymetric data to generate a cross section for the selected Nearshore Site. The bathymetry data collected for the embayment at the Nearshore Site fill a gap in our understanding of the interface for groundwater and surface-water exchange (fig. 14). The cross section shows the large tidal variability in hydraulic head for different tidal stages for the deep aquifer (fig. 15); the cross section in figure 15 uses the same potentiometric surfaces shown in figure 12. For the shallow aquifer, the tidal variability is minimal. The geometry of the potentiometric surfaces between the nearshore-most well and the shoreline is complex. The potentiometric surface of the deep aquifer probably slopes in a near-linear fashion toward the respective water level in the Duwamish River. The potentiometric surface of the shallow aquifer, however, is less well understood and likely depends on the extent of the confining unit that underlies the shallow aquifer. If the confining unit extends to, or very near to, the shoreline, then the potentiometric surface would likely parallel the near-horizontal orientation of the confining unit and water in this aquifer would emerge as a seep at the shoreline. If the confining unit is truncated between the closest groundwater well and the shoreline—which may be the case at this site because the path of the historical Duwamish River overlaps the easternmost terrestrial surface and may have eroded the confining layer—then the potentiometric surfaces of the shallow aquifer may trend more toward the respective water level in the Duwamish River. The nearshore geometry of the potentiometric surfaces is further confounded by intermittent shoreline armoring and other buried infrastructure, which could serve as either a barrier or a conduit to flow.
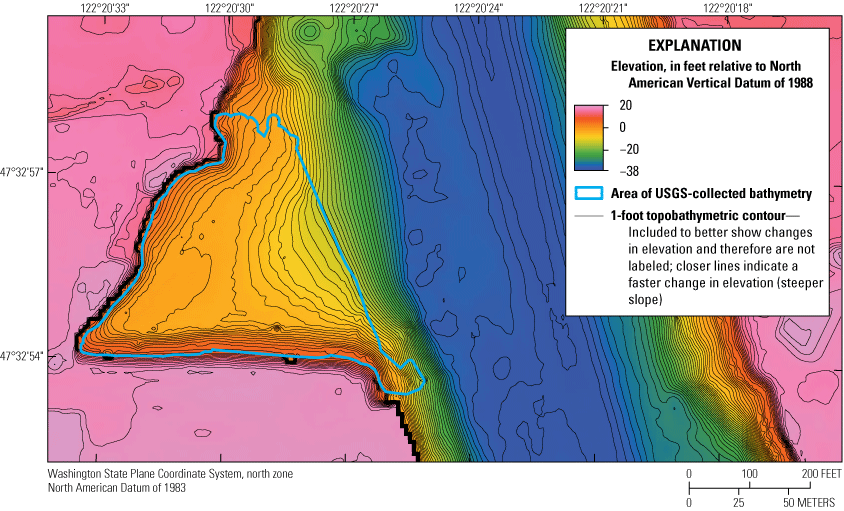
Map showing topobathymetric data for the selected Nearshore Site along the lower Duwamish Waterway, Seattle, Washington. Altitude data are from Lower Duwamish Waterway Group (2004), U.S. Geological Survey (2023a), and Mitchell and Opatz (2024).
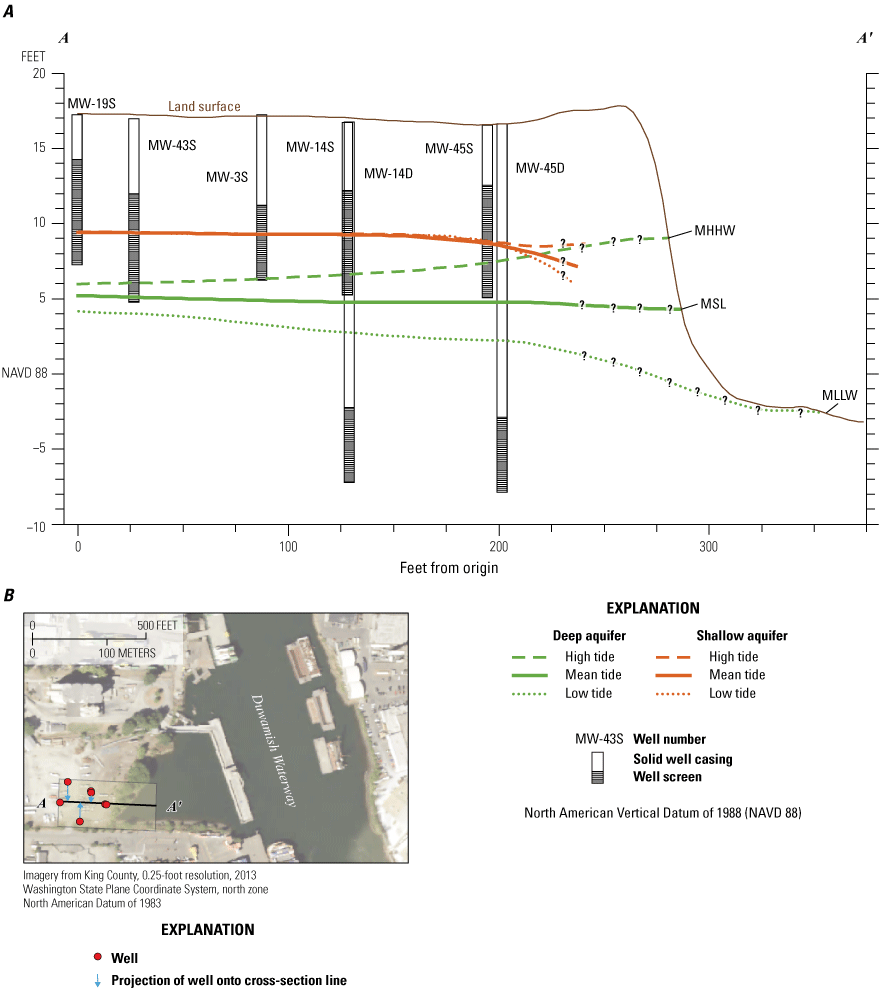
Cross section (A) and aerial image (B) showing U.S. Geological Survey monitoring wells and potentiometric surfaces developed for the selected Nearshore Site for the shallow and deep aquifers during low, mean, and high tides. Groundwater wells were projected onto the cross section and their water levels may not perfectly align with the potentiometric surfaces, which were developed with water-level data from additional wells. Potentiometric surfaces shown are identical to those in figure 12. The nearshore-most sections of the shallow potentiometric surfaces are omitted because of uncertainty related to their geometry near the shoreline. Uncertainty regarding the potentiometric surface of the deep aquifer near the shoreline is also indicated by question marks. The potentiometric surface of the shallow aquifer near the shore is not shown or estimated because it is poorly understood at this site.
Temperature
Temperature data recorded at the eight monitoring sites reveal mostly predictable trends (fig. 16). The water temperature in the Duwamish River had a strong seasonal signal, from a low of about 7 °C in the winter to a high of about 15 °C in the summer. The shallow monitoring wells had a seasonal pattern that peaked in autumn, which represents a 2–3 month lag behind the air-surface temperature (Smith and others, 2023); temperatures varied from about 11 to 17 °C. In contrast, the deep wells had a more attenuated temperature range—about 13–14 °C, if the spikes in the record at MW-45D are omitted—and had a seasonal pattern representing a nearly 6-month lag behind the temperature in the Duwamish River. The asynchronous relation between temperatures in the Duwamish River and the deep wells is likely related to the time associated with heat transport (Anderson, 2005). Slight tidal variability is observed in the temperature data from the deep wells: about 0.1–0.3 °C. No tidal variability is observed in the temperature data from the shallow wells.
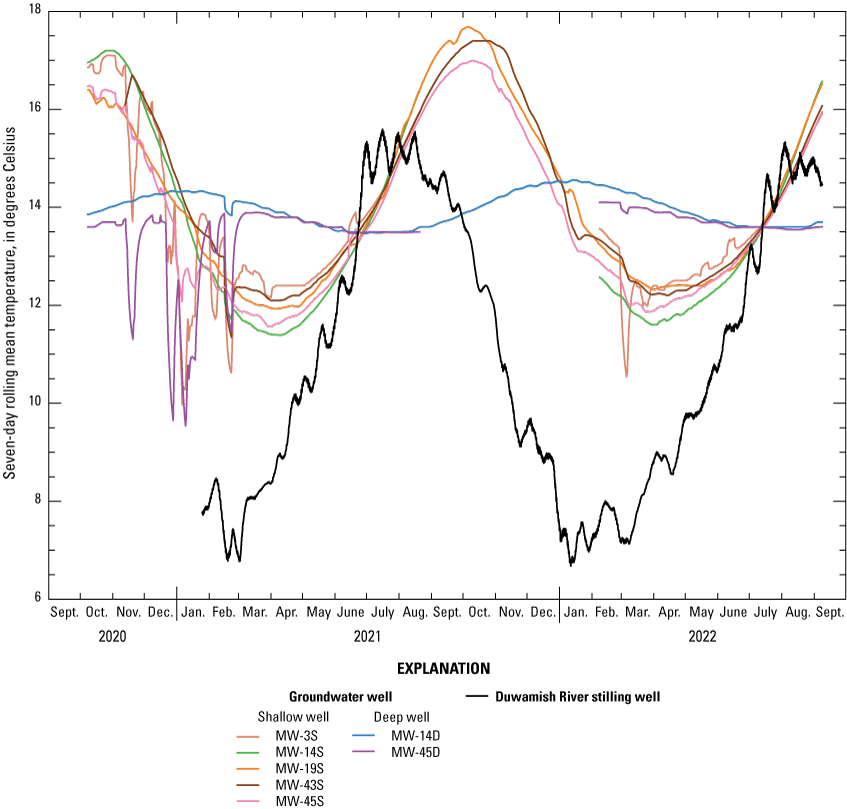
Graph showing 7-day rolling mean water temperatures recorded at monitoring locations at the selected Nearshore Site along the lower Duwamish Waterway, Seattle, Washington, 2020–22.
During winter, several prolonged decreases in the water temperature in the shallow and deep wells may be related to heavy precipitation. The wells were flush-mounted and overland runoff may have infiltrated the wells; alternatively, the signal could reflect a large flux of colder groundwater flowing past the wells from upland recharge.
Specific Conductance
Specific-conductance data at all eight monitoring locations indicate greater variability and less predictability than either water level or temperature data (fig. 17). The Duwamish River has a slight seasonal signal, with a peak in summer that corresponds with decreased influx from the Green River, and thus less fresh water to displace salt water entering from Puget Sound. Daily to monthly variations that correspond to the interplay of tidal conditions and river discharge are superimposed on the rough seasonal signal. The specific-conductance data from the groundwater wells have no clear seasonal signal and the records contain many abrupt decreases. Much like the temperature data discussed in section, “Temperature,” it is unclear whether these abrupt decreases represent overland runoff infiltrating the wells or a large flux of fresher groundwater flowing past the wells from upland recharge. The deep wells have a specific conductance that is consistently greater than the shallow wells. Specific conductance at the shallow wells ranges from about 250 to 750 µS/cm, whereas the specific conductance at the deep wells ranges from about 1,000 to 1,750 µS/cm. It is unclear why the specific conductance values observed at the two deep wells (MW-14D and MW-45D) are dissimilar in both pattern and amplitude, but it may be related to overland infiltration or subsurface heterogeneity.
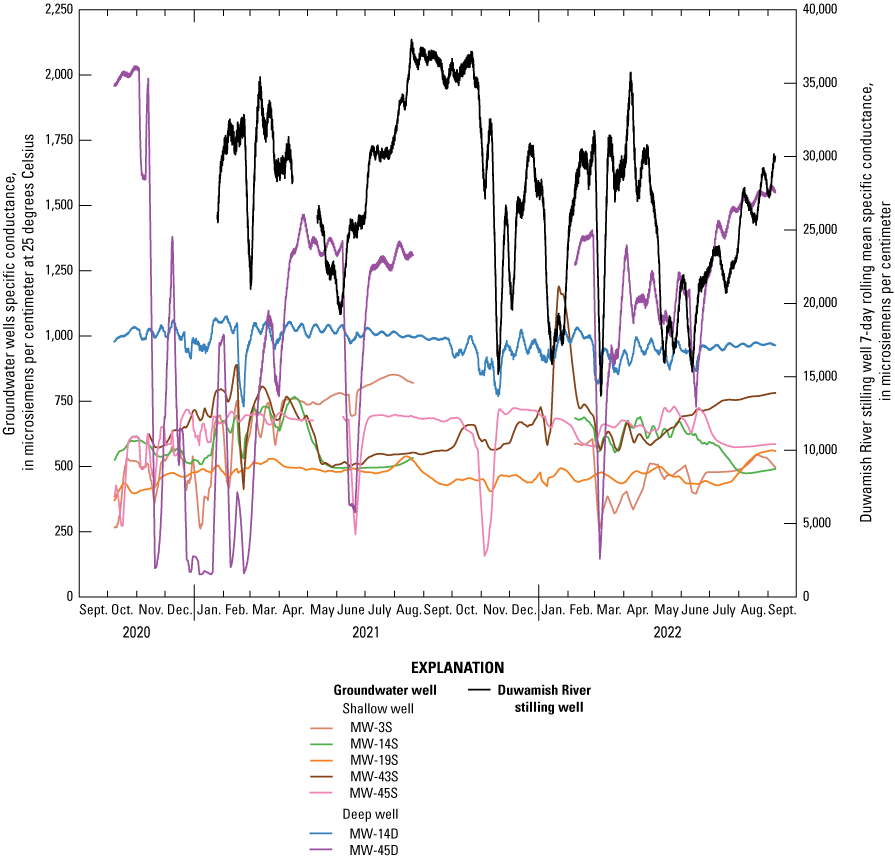
Graph showing 7-day rolling mean specific conductance recorded at monitoring locations at the selected Nearshore Site along the lower Duwamish Waterway, Seattle, Washington, 2020–22.
Tidal variability of the specific-conductance data was observed in the deep wells and the Duwamish River; the shallow wells had no discernable tidal variation (fig. 9). The deep wells had a specific-conductance signal that lagged the Duwamish River by about 1 hour (consistent with the short-term tidal lags of water level and temperature). The typical 25-hour tidal range of specific conductance observed in the Duwamish River stilling well was about 25,000–35,000 µS/cm. The specific-conductance sensor for the Duwamish River stilling well was at an altitude of about −4 ft relative to NAVD 88; for comparison, the mean lower low water (MLLW) and mean higher high water (MHHW) at this location during the about 2 years of data collection were 8.94 and −2.42 ft, respectively, and the lowest altitude of the Duwamish River in the river’s cross section at this location was about −37 ft. That is, the specific-conductance data recorded by the sensor represent the salinity at a point that was about one-third of the total river depth at this cross section. Water above the sensor is presumably of lower specific conductance, and water lower than the sensor in the river is presumably of higher specific conductance.
Regional Conceptual Groundwater Model
A conceptual groundwater model is a simplified description of the major inflow and outflow sources and transmission and storage properties for a groundwater-flow system. The conceptual model presented here builds upon that of Woodward and others (1995) and Booth and Herman (1998) by incorporating information from several recent site-specific remedial-investigation studies conducted in the lower Duwamish River valley, although the general ideas presented in these original reports have not been substantially changed.
Groundwater Occurrence and Movement
The relation between recharge and discharge plays a key role in the regional groundwater system because, in broad terms, groundwater moves from recharge zones to discharge zones. The uplands that surround the valley are regional recharge areas whereas the valley itself is a regional discharge area, owing to its low altitude. Recharge occurs when water percolates downward to the water table or is otherwise added to the groundwater system. The primary sources of recharge in the lower Duwamish River valley are the direct infiltration of precipitation and lateral flow from the adjacent aquifer(s) in the uplands; less prominent sources include infiltration from surface-water bodies (for example, lakes, streams, and wetlands) and leakage from water-distribution pipes. Woodward and others (1995) used the deep-percolation model (Bauer and Vaccaro, 1987) to estimate recharge for several basins in King County; although the lower Duwamish River valley was not among the basins that were modeled, the adjacent basins provide the best available estimate of recharge for the area—about 5–20 inches per year. The understanding of recharge in the lower Duwamish River valley is complicated by the large area of impervious surfaces, which enable increased evaporation, and the corresponding storm-drainage systems may route precipitation directly to surface-water bodies.
Groundwater in the lower Duwamish River valley is generally described as a single aquifer, although there may be local variations to the groundwater conditions. The aquifer is primarily contained within alluvial deposits and in some places extends into the overlying fill materials. The valley aquifer is bounded laterally by glacial and non-glacial deposits of the uplands; although not disconnected, the valley and adjacent uplands have distinct hydrogeologic regimes and are typically treated separately. The aquifer is bounded on the bottom by a sequence of glacial and non-glacial deposits with varying thickness and, ultimately, less permeable bedrock. Aquifer thickness ranges from about 30 to 100 ft (Booth and Herman, 1998). The depth to water is generally 10 ft or less throughout the river valley.
Groundwater in the aquifer occurs under unconfined, semi-confined, and confined conditions. The first water encountered with depth is generally unconfined. Lenses of fine-grained silts and clays as much as about 4-ft thick, generally representing tideflats or dredged fill deposits, are common throughout the valley and often overlie semi-confined or confined groundwater. When they occur near the land surface, the silt and clay lenses can also support thin perched aquifers that offer some separation between the shallowest layer of groundwater and the more extensive groundwater system below. The low-permeability lenses, although common, seldom are laterally extensive and are often reported as discontinuous or patchy.
Regional groundwater flow of the water table is from the valley uplands to the valley floor and the Duwamish River (fig. 18). Parallel to the Duwamish River, the west and east uplands have a groundwater divide where groundwater flows either to the Duwamish River or the opposite water body (that is, Puget Sound and Lake Washington, respectively). Water levels in the uplands range from about 50 to more than 400 ft, whereas water levels in the valley are generally 5–10 ft. The water-level gradient is steep in the valley walls separating the uplands from the valley floor, particularly on the western side.
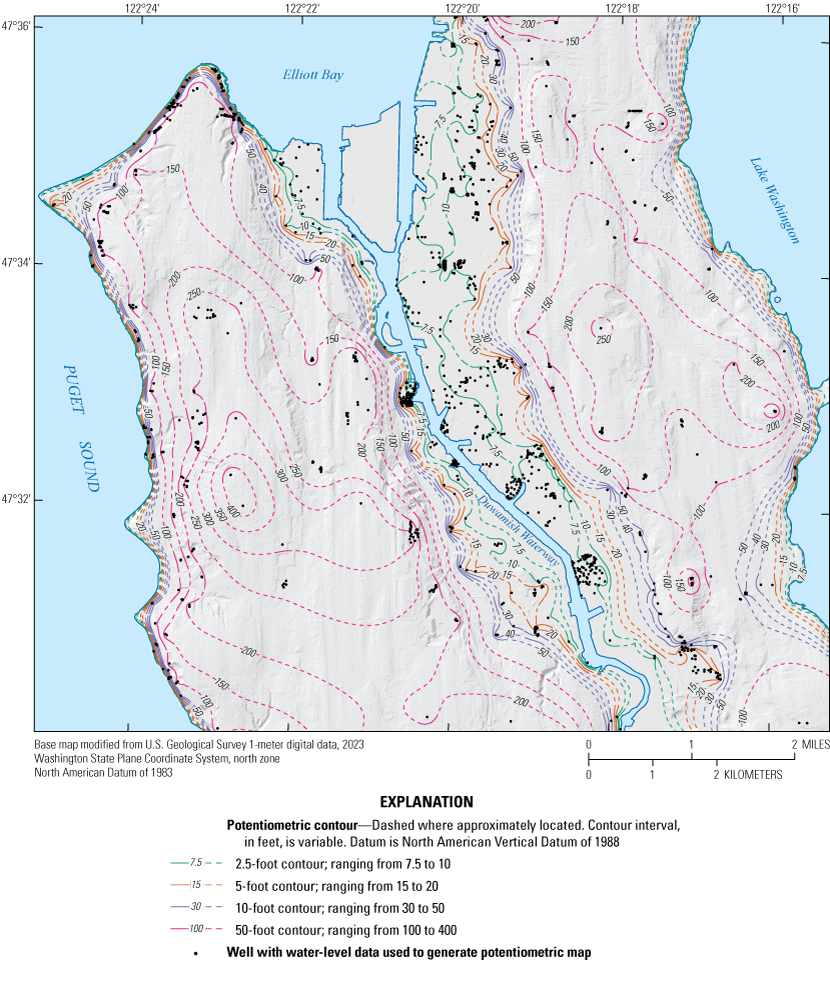
Map showing potentiometric surface for the groundwater table in the lower Duwamish River valley, Seattle, Washington.
Groundwater discharge occurs as base flow to streams and as springflow, seepage along bluffs, withdrawals from wells, evapotranspiration, and submarine groundwater discharge (along the coast of Puget Sound and shoreline of Lake Washington; Woodward and others, 1995). As groundwater moves from recharge areas to stream discharge zones, it generally has an upward vertical component as it approaches the discharge zone—in this case, the Duwamish River. The typical upward gradient of groundwater flow at discharge zones is further expected in the lower Duwamish River valley because of the presence of the salt wedge, which, in addition to its longitudinal extent up the river, extends laterally into the nearshore terrestrial environment (fig. 19). Owing to its greater density, salt water sinks into the ground and underlies the riverbed in the lower reaches of the Duwamish River—where the salt wedge is present—and migrates into the nearshore sediments. At the contact between the salt wedge and the fresh groundwater in the aquifer, some degree of mixing and diffusion occurs, creating a transition zone between the two endmembers. As fresh groundwater flows toward the river, the density contrast between the fresh groundwater and the salty groundwater forces most of the fresh groundwater to flow above the mixing zone and salt wedge and discharge at the shoreline. At high tide, the saline water in the Duwamish River intrudes into the adjacent aquifer and forms an upper saline cell near the shoreline. The upper saline cell may further constrain the area through which fresh water can discharge to the river at low tide; at each high tide, the upper saline cell will be replenished with saline water from the river (for example, see fig. 19C–D).
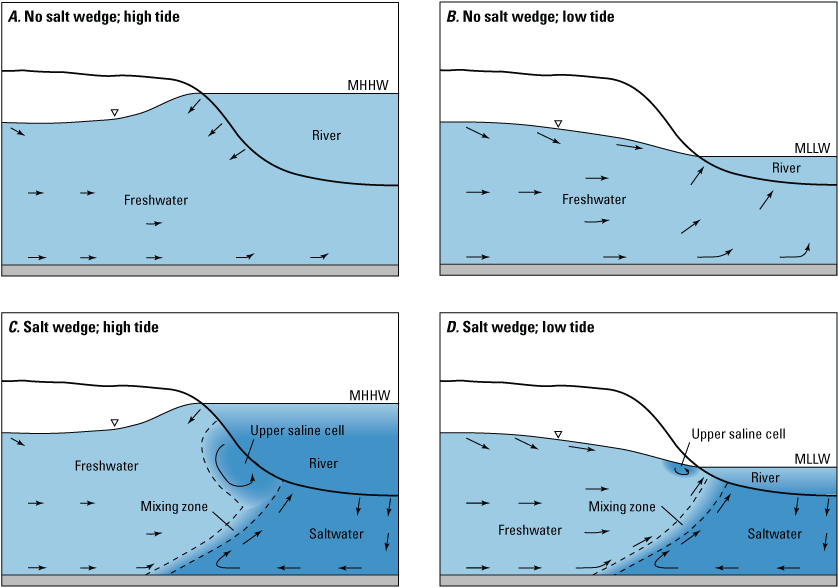
Conceptual diagrams showing cross sections of groundwater and surface-water interactions between a groundwater system and the adjacent tidal river under different conditions. (A) No salt wedge in the river during high tide. (B) No salt wedge in the river during low tide. (C) Salt wedge present during high tide. (D) Salt wedge present in the river during low tide. MLLW, mean lower low water; MHHW, mean higher high water.
The large tidal range of the Duwamish River—averaging about 11.4 ft and sometimes exceeding 16 ft—introduces further complexity into the groundwater-discharge regime. At high tide, the river stage exceeds the nearshore groundwater level; thus, the typical flow gradient reverses and river water and the nearshore groundwater flow inland (for example, see fig. 11). The net movement of groundwater remains toward the river over each tidal cycle. In the lower reaches of the river, much of the river water entering the terrestrial nearshore environment is brackish or highly saline (for example, 5,000–50,000 µS/cm). As saline river water flows inland at high tide, it mixes with groundwater through diffusion and dispersion, then flows back toward the river at low tide. The inland penetration of salt water is likely to be irregular, owing to the heterogeneity in the nearshore environment. In some places, a recirculating upper saline cell may form in the nearshore environment between MLLW and MHHW. The presence of an upper saline cell would provide a constraint on the groundwater flow path to the river in addition to the underlying salt wedge (fig. 19).
Hydraulic Properties
The distribution and variability of hydraulic properties of the aquifer materials govern the storage and transport of groundwater. The alluvial aquifer in the lower Duwamish River valley occurs within sand and silty sand, in addition to some shallower fill material. Hydraulic conductivity has been estimated as part of several site-specific remedial-investigation studies; the two most common methods are slug tests and tidal analysis of water levels. Estimates of hydraulic conductivity range from about 1 to 750 ft per day (ft/d; Anchor, Q.E.A., LLC., 2019; GeoEngineers, Inc., 2019)—although most estimates are less than 100 ft/d—and results vary based on the depth of investigation and other local-scale geologic heterogeneities. At the Nearshore Site with USGS data collection, the hydraulic conductivity in the shallow aquifer, determined using slug tests, was about 3.5 ft/d (ERM-West, Inc., 2020).
Horizontal hydraulic gradients determined in the lower Duwamish River valley generally range from 0.001 to 0.01 ft/ft. Using the 2 years of data collected at the Nearshore Site, the horizontal gradient in the deeper aquifer between MW-14D and MW-45D ranged from −0.03 to 0.03 ft/ft—representing the gradient reversal due to the tides—and averaged about 0.0006 ft/ft. In the shallow aquifer, the hydraulic gradient—as computed using the data from MW-3S, MW-14S, MW-19S, MW-43S, and MW-45S—ranged from 0.006 to 0.008 ft/ft. Assuming a porosity of 0.25, a hydraulic conductivity of about 3.5 ft/d, and a horizontal gradient of 0.007 ft/ft, the average linear flow velocity would be about 36 ft per year.
Vertical gradients in the lower Duwamish River valley range from about 0.002 to 0.7 ft/ft and vary based on depth and location. In general, shallow wells indicate a downward vertical gradient and deeper wells indicate an upward direction. Contrary to the conceptual understanding of groundwater flow as it approaches the river, there is no clear pattern in the available data showing a trend toward an upward vertical gradient; this may be a consequence of the depth of investigation or it may indicate that the zone of upward groundwater flow near the river is limited to a narrow zone near the shoreline where wells and data may be sparse. The vertical gradient at the shallow/deep well pairs (MW-14S and MW-14D; MW-45S and MW-45D) ranged from about 0.3 to 0.5 ft/ft in the downward direction. The west side of the Duwamish River generally has larger vertical gradients than the east side, potentially because more groundwater flows into the river valley from the western uplands than the eastern uplands.
Groundwater and Surface-Water Interactions
Characteristics of the Nearshore Mixing Zone
As groundwater flow approaches a receiving surface-water body, the flow paths—and contaminant-transport pathways and mechanisms—become complex owing to the interaction with infiltrating surface water. Groundwater in coastal aquifers discharges to receiving surface waters through advective and dispersive processes. Flow paths and discharge rates are controlled by meteorology, aquifer properties (porosity, hydraulic gradient, and hydraulic conductivity), shoreline conditions, and receiving-water hydrology. The receiving surface water can also infiltrate into the shoreline materials during periods of high water-level altitudes such as flooding, regulated releases of water, and high tides.
In coastal zones, where the receiving surface water is salt water, the salt water forms a classical salt-wedge shape, with the toe of the salt wedge extending below the less dense fresh water. The salt wedge extends longitudinally up the river (as described in section, “Duwamish River Characteristics”) and also infiltrates laterally into the saturated aquifer materials. Because of the similarities to the hydrology of a surface-water estuary, this subsurface flow regime is often referred to as a subterranean estuary (Moore, 1999). This dual-density, dual-flow regime has been extensively modeled (for example, Li and others, 2003; Robinson and others, 2018), and simulations typically identify a sharp gradient between the net seaward-moving fresh groundwater and underlying infiltrating seawater (fig. 19).
Above the salt wedge, in the shallow intertidal zone, sub-daily tidal oscillations induce hydraulic gradients that drive seawater circulation through the sediment, forming an upper recirculating seawater cell, or upper saline cell (fig. 19). This subsurface interface between discharging groundwater and infiltrating surface water is a highly dynamic mixing zone. The shape and extent of the mixing zone can vary in time and space and is impacted by tidal forcing and other hydrogeologic characteristics, including shoreline slope, and shoreline and aquifer porosity. The mixing zone may be small or non-existent in areas with impermeable shoreline barriers, where the beach slope is very shallow, or where the groundwater flux is high (Evans and Wilson, 2016). Conversely, the mixing zone may be large in areas with coarse beach substrate and high porosity, steep beach slope, or where groundwater flux is low.
The mixing zone is a region of dynamic hydrogeochemical conditions that can impact flow paths and composition of the discharging water (for example, Fritz and Arntzen, 2007). In addition to classical advective and dispersive processes that describe groundwater and associated solute flow, other processes can occur in the mixing zone, including dispersion owing to tidal pumping and wave run-up, and chemical transformations. Recirculating surface water in the mixing zone introduces oxygen to the aquifer materials, thereby modifying geochemical conditions (such as redox) and the amounts and types of organic matter, major ions, nutrients, and bacteria. These conditions, in turn, can modify the characteristics of contaminant transport; for example, the conditions under which sorption and biodegradation occur can be episodically or permanently altered. Furthermore, preferential flow paths can exist that route fresh groundwater directly to the receiving surface water (for example, some groundwater seeps), or, conversely, allow seawater to infiltrate farther inland than the mixing zone and interact with previously uncontacted aquifer materials. Because of these spatial, temporal, and physicochemical dynamics, modeling and measurement of the groundwater and surface-water mixing zone is complex.
Nearshore Characteristics of the Lower Duwamish Waterway
Groundwater and surface-water interactions in the lower Duwamish Waterway are complex owing to three overarching factors. First, the receiving surface water has a water-level range attributable to sub-daily, monthly, and seasonal tidal cycles in the estuary and regulated river flows for flood control and dam operation (with instantaneous discharge of water at the Howard Hanson Dam that varies by more than an order of magnitude throughout the year)—the tidal range averages at least 11 ft and sometimes exceeds 16 ft.
Second, the receiving surface water has variable density and water quality. Regardless of tide and river-discharge conditions, the salt wedge is always present in the lower Duwamish Waterway seaward of RM 1.9 (McKeon and others, 2020). Even in areas where the salt wedge is generally present, the thickness of the salt wedge, the thickness of the overlying freshwater lens, and the flow direction and velocity of both layers will vary at sub-daily time scales. Upstream from RM 1.9, the presence and extent of the salt wedge will vary relative to the overlying river water, meaning that at some times of the year the bank-infiltrating surface water will be mostly seawater and at other times it will be mostly fresh water. Seawater composition has high ionic strength (dominated by cations such as sodium, magnesium, and calcium) and anions such as chloride, fluoride, and sulfate. The composition of river water in the lower Duwamish Waterway is variable (for example, Conn and others, 2018) and can contain elevated metals, organics, and bacteria from upstream and adjacent sources such as stormwater outfalls or sediment resuspension.
The third complexity in the lower Duwamish Waterway is that the nearshore subsurface and shoreline conditions are heterogenous. The shoreline has been modified over many years with armor of varying permeability (for example, with sheet walls and rock), including some buried features (Morley and others, 2012). The dredged river-sediment materials that were removed from the river during its conversion into a waterway were often deposited on the adjacent lands above the natural aquifer. Since then, fill material of various grain sizes and gravel have been deposited above the dredge materials on many of the properties. In some locations, this has resulted in a shallow perched aquifer—fed primarily by infiltrating precipitation—above and hydrologically separated from the primary aquifer by a fine-grained confining layer. Within these aquifers, numerous contaminant plumes are present with varying size, depth, and concentration.
At the selected Nearshore Site, the shallow and deep aquifers are connected to the river to various degrees, as indicated by discharge from seeps at the shore face and discharge into the mixing zone at and below the sediment-water interface. The deeper natural aquifer has greater short-term variations because of its depth and increased discharge-dependence on tidal stage. This connectivity between the groundwater and river water and short-term tidal variability seen at the Nearshore Site is likely a general pattern throughout the lower Duwamish Waterway, although site-specific differences will affect where that connectivity occurs; for example, flow may be forced through cracks along an armored shoreline, flow may occur laterally along a natural shoreline, and flow may occur below an armored shoreline through diffuse subterranean discharge. Furthermore, the coarse and heterogenous substrate and the presence of urban subsurface infrastructure (for example, stormwater pipes and outfalls) likely result in bi-directional preferential flow paths of fresh groundwater discharge and surface-water infiltration that are difficult to locate and model.
Based on the data compiled for this study, the mixing zone in the lower Duwamish Waterway appears generally limited to tens to a few hundred feet inland from the shoreline. For example, specific conductance (an easily measured proxy for salinity) was slightly elevated in the deep monitoring wells at the Nearshore Site (about 1,000–1,500 µS/cm) relative to shallow monitoring wells (less than 500 µS/cm) but was well below seawater values (greater than 40,000 µS/cm). This suggests that tidal forcing of hydraulic head extends a few hundred feet inland but, in general, the salt water itself does not extend this far. Some exceptions exist, where specific conductance was elevated (median specific conductance greater than 5,000 µS/cm) in monitoring wells as far inland as about 400 ft (fig. 20). Additional monitoring is needed to confirm these values and clarify the hydrogeologic conditions resulting in their occurrence to determine if these locations also could preferentially transport contaminants to the lower Duwamish Waterway. There is not enough existing data, especially in the nearshore, to fully characterize the mixing zone shape and extent throughout the lower Duwamish Waterway and understand seasonal impacts.
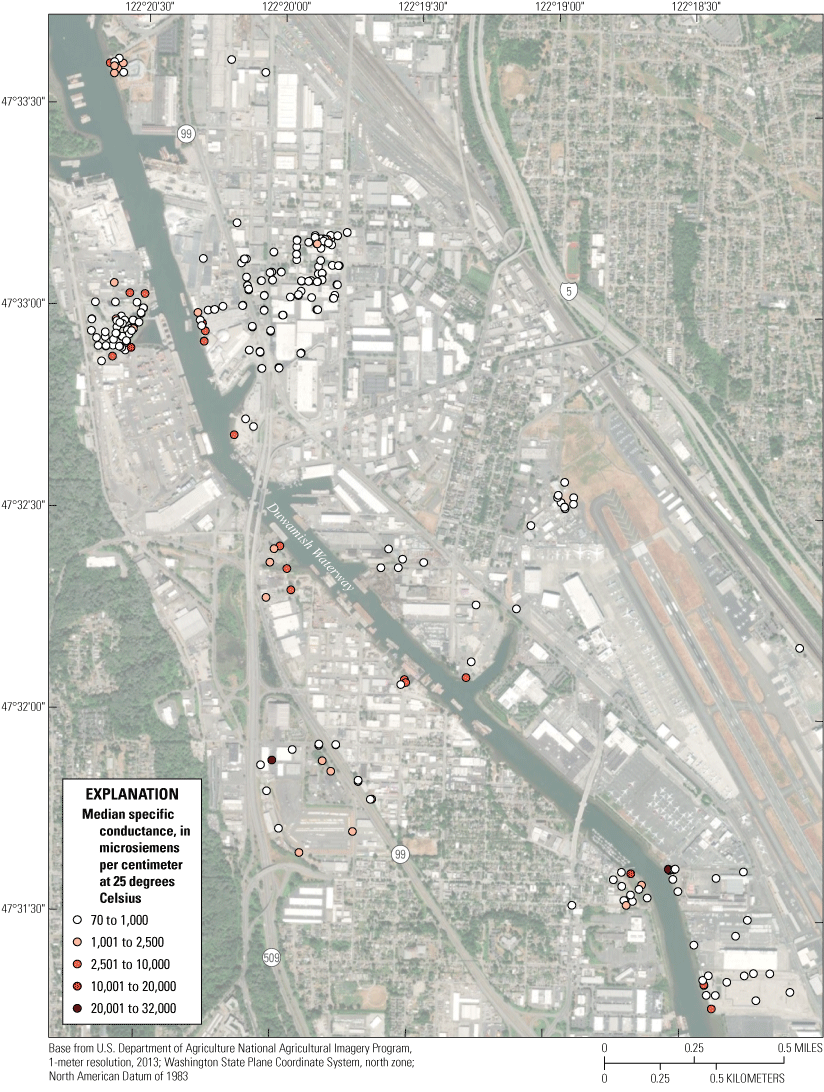
Map showing the median specific conductance from discrete measurements of groundwater at monitoring wells in the lower Duwamish Waterway, Seattle, Washington. Data from U.S. Geological Survey (2023c) and Washington State Department of Ecology (2023d).
The nearshore groundwater level in the natural aquifer represents an attenuated version of the water-level conditions in the lower Duwamish Waterway. High river-discharge events are modulated as they move downstream in the watershed; the tidal signal increases relative to the river-discharge signal with distance downstream. For example, during December 20, 2020–January 20, 2021, there were successive increases in river flow (from precipitation and regulated releases of water) that rapidly increased the gage height at USGS streamgage 12113390 at RM 10.8 (fig. 21). At the next downstream streamgage, USGS streamgage 12113415 at RM 6.5, tidal forcing dominated over the high river flows; an increase in gage height independent of tide is visible only during the initial stage rise, after which the tidal signal, now raised above pre-storm levels, characterizes the hydrology (fig. 21). The hydrology at the Duwamish River stilling well, at RM 1.4 (fig. 21), is tidally dominant and has the full Puget Sound tidal magnitude of as much as about 15-ft swings between lower low tides and higher high tides. A nearshore monitoring well, MW-45D, within a few hundred feet of shoreline (fig. 21; fig. 6), closely mimics the tidal signal of the Duwamish River stilling well, with an attenuated tidal amplitude (of about 7 ft) and minimal tidal lag (about 1 hour). In contrast, the water level in the shallow, perched aquifer has minimal tidal influence (tide-induced oscillations of less than 0.1 ft) and its signal is primarily influenced by infiltrating precipitation from the land surface.
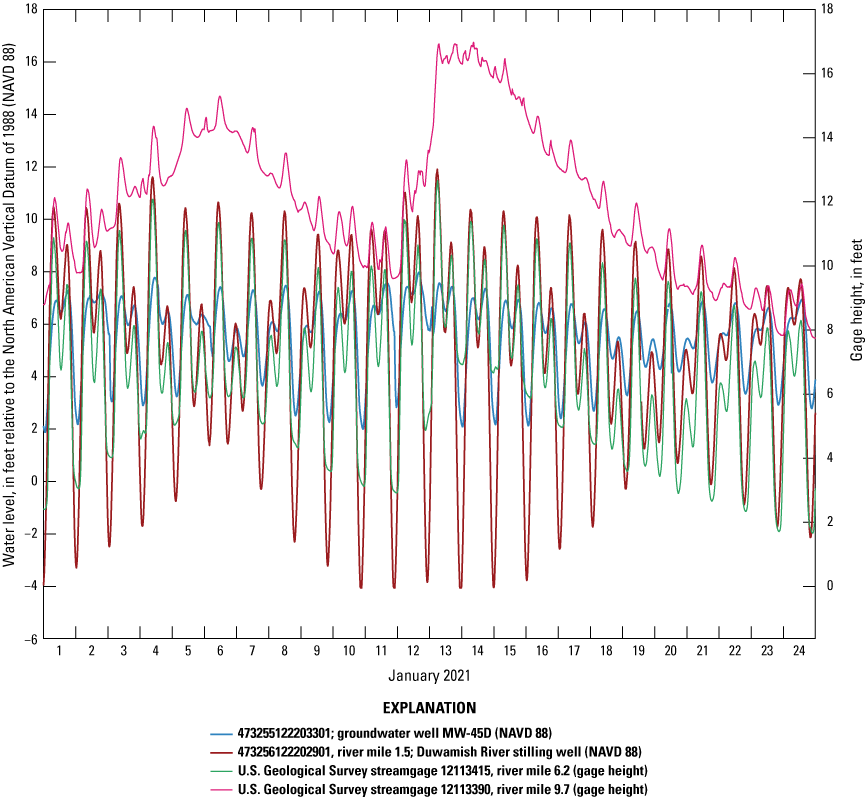
Hydrograph showing groundwater levels and river levels at four sites during a combined precipitation event and regulated river release, Duwamish River, Seattle, Washington, January 1–24, 2021. The precipitation event and regulated river release are indicated by the rise in gage height at U.S. Geological Survey streamgage 12113390.
Tidal Effects on Contaminant Transport
Terrestrial contaminant plumes originating inland of the tidal mixing zone are transported with groundwater by advective and dispersive mechanisms and generally discharge to the nearshore environment within the submarine fresh groundwater discharge zone (fig. 19). Previous modeling of contaminant transport in coastal aquifers (La Licata and others, 2007), including in Puget Sound (Yager and others, 2020), indicated that the tidal fluctuations had a small effect on the contaminant mass discharged to the receiving water because the period of tidal fluctuations (about 12-hour cycles) is rapid compared with groundwater velocities, which generally range from tens to hundreds of feet per year. However, these types of long-term modeling simulations with coarse grid sizes often cannot account for the dynamic hydrologic and biogeochemical complexities in the intertidal nearshore mixing zone that influence contaminant transport processes, concentrations, and fluxes.
Modeling simulations by Robinson and others (2007) indicated that the upper saline cell changes contaminant transport pathways and reduces (dilutes) concentrations and fluxes from aquifer to coastal water. Similarly, one-dimensional modeling indicated that tidal fluctuations diluted contaminant concentrations by the water infiltrating inland during high tide cycles within the mixing zone (Yim and Mohsen, 1992). In contrast, Li and others (1999) and Moore (1999) reported that tidal effects may increase chemical fluxes over short periods (such as monthly periods) and by a factor of as much as 20 times above the average level. One-dimensional modeling by Ding and Peng (2009) indicated that contaminant discharge can be enhanced by tides by a factor of 2 or 3 during early stages of discharge, and the enhanced discharge can still be substantial during later stages by 30–50 percent. None of these modeling exercises consider a scenario in which the contaminant plume is within the mixing zone itself and is subject to continuous and repeated cycles of infiltration and draining.
Dynamic redox conditions in the mixing zone (indicated by, for example, pH, redox potential, dissolved oxygen, and the presence and levels of ions such as iron and sulfate)—as well as dissolved organic carbon, nutrients, bacteria and aquifer sediment characteristics—strongly influence the role of contaminant sorption and transformation processes. In a field study of a dissolved hydrocarbon groundwater plume, Westbrook and others (2005) showed that contaminant distribution and spatial and temporal discharge were strongly influenced by the nearshore tidal effects in the mixing zone. They found that groundwater discharge occurred in a zone less than 33 ft (10 meters [m]) from the high-tide mark, and mostly less than 16 ft (5 m) from the shoreline. The leading front of the organic contaminant plume extended only about 8–16 ft (2.5–5 m) into the river sediments from the shoreline. The mixing zone created an active zone for aerobic bacterial populations and reduced hydrocarbon concentrations at the discharge point to the receiving surface water. This finding has been corroborated in model simulations for hydrocarbons (Robinson and others, 2009) as well as other aerobically biodegradable contaminants such as alkanes and PAHs (Geng and others, 2015). Few field studies have investigated the transport of toxic, persistent and bioaccumulative industrial contaminants that are not readily biodegraded, such as PCBs and dioxins/furans, in the nearshore mixing zone. Concentrations and fluxes of these compounds likely are controlled by the amount of dissolved and particulate organic carbon, to which they sorb, in the nearshore environment.
Inorganic chemical-transport mechanisms are also impacted in the mixing zone in complex ways, often correlated to redox, the presence of oxides like iron, aluminum, and manganese, and sulfur and carbon. Yu and others (2023) found that arsenic and iron in groundwater were immobilized onto near-bank sediment when the reducing groundwater interacted with oxic water in the mixing zone. Conversely, arsenic sorbed to nearshore unsaturated oxic sediments can be mobilized during redox transitions caused by saturation of oxic sediments (Polizzotto and others, 2008). When the saturating water has a high salinity, the increase in ionic strength can result in increased acidity, as indicated by lower pH and higher alkalinity, and mobilization of exchangeable trace metals. The dominant cations in seawater (sodium, calcium, and magnesium) compete with and displace other cationic metals from negatively charged sediment surfaces (Wong and others, 2010). Studies have reported increased mobilization of cationic metals such as nickel, cadmium (Millward and Liu, 2003), zinc (Hahne and Kroontje, 1973), and mercury (Tiffreau and others, 1995; Grassi and Netti, 2000), forming dissolved chloro-complexes in seawater within seawater mixing zones. Furthermore, a “salting out” effect has been shown for the flame retardant perfluorooctane sulfonate (PFOS), attributed to a reduction of aqueous solubility in seawater and apparent increase in sorption coefficient (Li and others, 2022). Contaminant transport will be site-specific and temporally variable owing to complex interactions between contaminants and a suite of environmental variables.
Lower Duwamish Waterway Nearshore Contaminant Implications
In the long term (years), regional groundwater velocity—determined by hydraulic gradient and hydraulic conductivity—has the most significant influence on the contaminant-transport rate. But tidal effects are also important in areas immediately adjacent to the shoreline and play a role on relatively short time scales. In Puget Sound and the lower Duwamish Waterway, there are many instances of landfills, industrial-waste sites, and other contaminated sites within a few hundred feet of the shoreline or in the water at the shoreline. These sites are often situated in coarse, artificial, and heterogenous material. This fact, in combination with the large tidal range in Puget Sound, creates favorable conditions for contaminant exchange in the nearshore environment.
Few studies, however, have been conducted that quantify contaminant fluxes—or even nearshore water fluxes—owing, in part, to the difficulty in field monitoring in the nearshore mixing area. Rosenberry and others (2013, p. 2,982) measured large and highly variable nearshore seepage rates in a Puget Sound intertidal zone and concluded that “volumes of water and associated nutrients and chemicals that flow across the sediment-water interface each day are much larger than previously thought (Simonds and others, 2008), resulting in a substantially larger mixing volume in the pore waters beneath the broad intertidal zone...” Estimates of groundwater flux to the nearshore based on net fresh groundwater discharge only—without accounting for subterranean seawater exchange or recirculating water in the intertidal, mixing zone—may greatly underestimate actual water fluxes.
The heterogenous aquifer properties in the lower Duwamish Waterway will contribute to spatially and temporally dynamic contaminant-transport processes. Most existing literature (modeling and field measurements) assumes a relatively shallow contaminant plume some distance inland that is transported in groundwater to the nearshore environment. In the lower Duwamish Waterway, contaminant plumes may be present (1) in shallow, unsaturated zones primarily impacted by infiltrating precipitation; (2) in deeper, saturated aquifer materials that interact with the lower Duwamish Waterway subterranean estuary; and (3) at various horizontal distances inland from the lower Duwamish Waterway, including within the dynamic nearshore mixing zone. Contaminant travel times and fluxes to the lower Duwamish Waterway will vary between these different zones and may be, for example, on the order of years within the freshgroundwater system but much faster within the mixing zone and along preferential flow paths where contaminants can be transported to the nearshore quickly with minimal attenuation. Therefore, assessing contaminant transport from nearshore sites to the lower Duwamish Waterway requires overlaying contaminant plumes with hydrogeologic conditions including, and perhaps most importantly, in the poorly understood nearshore mixing zone.
Water temperature is a common tool in groundwater and surface-water interaction studies because strong seasonal contrasts in temperature enable the ready distinction between water types—groundwater typically is colder than surface water in summer and warmer than surface water in winter. In the lower Duwamish Waterway, however, there are three (or more) endmembers rather than two: (1) groundwater (from the natural aquifer and possibly from the shallow, perched aquifer), (2) Puget Sound seawater, and (3) river water (composed of a mixture of upper watershed runoff and stormwater discharges). The temperature profile in each of these endmembers varies spatially and temporally, complicating the interpretation of temperature data as a tool for understanding groundwater and surface-water interactions. Furthermore, it can be difficult to identify contaminant sources in the nearshore mixing zone when both groundwater and nearshore sediment are historically contaminated, as is the case in many parts of the lower Duwamish Waterway. A toolbox of environmental and chemical tracers can be used in contaminant sourcing studies. Data and modeling studies would help bring better understanding of the role of groundwater and surface-water interactions in contaminant transport to the lower Duwamish Waterway and, therefore, would support ongoing clean-up activities in the basin for improved ecosystem and human health.
Data Gaps and Uncertainties
In the current study, existing data were compiled from multiple sources collected over different time periods and integrated with new USGS data collection. The current study identified numerous data gaps and uncertainties, listed below, regarding groundwater and surface-water interactions and implications for contaminant transport in the lower Duwamish Waterway:
-
1. A more complete regional groundwater-level monitoring network would confirm previous values and fill in spatial and temporal gaps.
-
2. Inclusion of a simple proxy for salinity, such as specific conductance, in the above monitoring network would further characterize the inland extent of salt water and the upstream variability.
-
3. The most informative monitoring would cover the range of expected conditions to identify any seasonal differences in the shape and extent of the mixing zone. Examples include extreme hydrologic events such as (a) a winter high tide coincident with a precipitation- and (or) regulated release-induced high river flow, or (b) a summer low tide coincident with low annual river flows.
-
4. A monitoring network that includes deep wells (existing or new) would help to identify the inland location of the subterranean salt wedge. Most existing groundwater data are from relatively shallow monitoring wells (less than 50 ft deep) with fresh or brackish conditions. Additionally, geophysical tools such as electromagnetic induction and (or) electrical resistivity tomography can be used to evaluate the shape and extent of the salt wedge.
-
5. Monitoring wells closer to the shoreline would help to improve the understanding of mixing-zone characteristics. Existing monitoring wells are mostly inland of the mixing zone; thus, the understanding of flow and solute transport in the mixing zone is currently interpolated between measurements in more inland monitoring wells and in the lower Duwamish Waterway. This characterization could include the extent and depth of buried shoreline armoring and the presence and continuity of any confining layers. For example, the historical river path crossed the eastmost edge of the Nearshore Site in this study, which may suggest that the confining unit is truncated before reaching the current shoreline.
-
6. The prevalence of tidally controlled recirculation cells in the nearshore lower Duwamish Waterway, including their extent and geometry, is poorly understood. In particular, how do shoreline armoring and steep banks constrain occurrence and geometry of recirculation cells?
-
7. Seeps are present in the lower Duwamish Waterway and limited previous sampling has identified the presence of contaminants such as heavy metals in some seeps (ERM-West, Inc., 2020). Monitoring of environmental tracers and contaminants of concern (for example, PCBs, dioxins/furans, PAHs, and PFOS) in porewater and seeps would help to fill data gaps between groundwater and surface-water concentrations. This would help clarify the relation of seep altitudes to the presence or absence of a confining layer. Physical measurements of nearshore seepage rates under varying river discharge and tidal conditions would be valuable. This information, in combination with nearshore chemical concentrations, can be used to calculate chemical fluxes to the lower Duwamish Waterway from fresh groundwater, deep saline water, and the mixing zone in the intertidal recirculating cell.
-
8. Owing to the broad heterogeneity in shallow subsurface material throughout the valley, it is difficult to extrapolate from any single site-specific study to other sites or to a regional understanding.
Summary
The U.S. Geological Survey (USGS), in cooperation with the Washington State Department of Ecology (Ecology), conducted a study to describe the current understanding of the regional groundwater system of the lower Duwamish River valley and groundwater and surface-water interactions in the lower Duwamish Waterway. The lower Duwamish Waterway is the final 5-mile reach of the Duwamish River before it empties into Elliott Bay in Puget Sound near Seattle, Washington. The reach was straightened in the early 1900s and is maintained through regular dredging as a navigable waterway. The adjacent floodplains were filled with dredged river sediment and imported artificial fill material to support numerous industrial uses, including manufacturing, warehousing, and marinas, as well as residential development. Current and historical human activities have contaminated sediments within the waterway and the adjacent groundwater system. During 2001–02, the U.S. Environmental Protection Agency (EPA) and Ecology required remedial investigations and feasibility studies on the lower Duwamish Waterway pursuant to the Comprehensive Environmental Response, Compensation, and Liability Act (also known the “Superfund” program; Public Law 96-510) and the Washington Model Toxics Control Act (Revised Code of Washington, Chapter 70A.305) because of concerns about human health risks from exposure to contaminated sediments.
Available information was compiled and new data were collected to describe the regional groundwater system and site-specific groundwater and surface-water interactions. Owing to the EPA Superfund status of the lower Duwamish Waterway, many site-specific hydrogeologic investigations have been completed in the area by various consultants and organizations, in addition to a smaller number of regional studies. A nearshore site (hereinafter referred to as “Nearshore Site” to distinguish the particular site from general discussions of nearshore areas) along the western shoreline of the Duwamish River between river miles (RMs) 1.4 and 1.5 was selected for focused groundwater data collection by USGS. Data loggers were deployed in 7 groundwater wells and 1 stilling well in the Duwamish River to measure specific conductance, temperature, and depth at 15-minute intervals for a period of about 2 years. A bathymetric survey was completed to understand the interface of groundwater and surface-water interactions at a Nearshore Site where new groundwater data were being collected.
At the Nearshore Site, water levels in the shallow wells are 3–8 feet (ft) higher than water levels in the deep wells, providing evidence for a lack of a connection between the shallow and deep aquifers in this area. The shallow wells have a pronounced seasonal variability, with high water levels in winter and low water levels in summer; the deep wells show far less seasonal variability, with slight increases in winter and a near constant water level from spring to autumn. For the two sets of paired shallow/deep wells (MW-14S and MW-14D; MW-45S and MW-45D), the vertical hydraulic gradient ranged from about −0.3 to −0.4 foot per foot, indicating flow in the downward direction (that is, from the shallow zone to the deeper zone). The deep wells have a strong hydraulic connection to the Duwamish River, as evidenced by the synchronous water-level variability during a tidal cycle. A tidal response is present in the water-level records of the shallow wells closest to the river; however, the tidal variation is only about 0.01–0.03 ft and is barely perceptible. The potentiometric maps developed for the Nearshore Site and surrounding areas indicate large differences in groundwater-flow directions for the shallow and deep aquifers at low and high tides. For the shallow aquifer, flow is toward the lower Duwamish Waterway near the Nearshore Site, regardless of the tidal condition. For the deep aquifer, a potentiometric trough forms parallel to the shoreline during high tide, indicating that groundwater flow converges from the uplands to the west and the Duwamish River to the east. The geometry of the potentiometric surfaces between the nearshore-most well and the shoreline is complex and is further confounded by intermittent shoreline armoring and other buried infrastructure, which could serve as either a barrier or a conduit to flow.
Temperature data recorded at the eight monitoring sites reveal mostly predictable trends. The water temperature in the Duwamish River had a strong seasonal signal, from a low of about 7 degrees Celsius (°C) in winter to a high of about 15 °C in summer. The shallow monitoring wells had a seasonal pattern that peaked in autumn, which represents a 2–3 month lag behind the air-surface temperature (Smith and others, 2023); temperatures varied from about 11 to 17 °C. In contrast, the deep wells had a more attenuated temperature range—about 13–14 °C, if the spikes in the record at MW-45D are omitted—and had a seasonal pattern representing a nearly 6-month lag behind the temperature in the Duwamish River. Specific-conductance data at all eight monitoring locations have greater variability and less predictability than either water level or temperature. The Duwamish River has a slight seasonal signal, with a peak in summer that corresponds with decreased influx from the tributary Green River, and thus less fresh water to displace salt water entering from Puget Sound. Daily to monthly variations that correspond to the interplay of tidal conditions and river discharge are superimposed on the rough seasonal signal. The specific-conductance data from the groundwater wells have no clear seasonal signal and the records contain many abrupt decreases. Temperature and specific-conductance data are unclear as to whether these abrupt decreases represent overland runoff infiltrating the wells or a large flux of fresher groundwater flowing past the wells from upland recharge. The deep wells have a specific conductance that is consistently greater than the shallow wells. Specific conductance at the shallow wells ranges from about 250 to 750 µS/cm, whereas the specific conductance at the deep wells ranges from about 1,000 to 1,750 µS/cm.
The regional conceptual groundwater model for the lower Duwamish River valley remains largely unchanged from that described in previous work (for example, Woodward and others [1995] and Booth and Herman [1998]). The groundwater system is generally described as a single aquifer, although there may be local variations to the groundwater conditions. The aquifer is primarily contained within the alluvial deposits and in some places extends into the overlying fill materials. Groundwater in the aquifer occurs under confined, semi-confined, and unconfined conditions. The first water encountered with depth is generally unconfined. Lenses of fine-grained silts and clays as much as about 4-ft thick, generally representing tideflats or dredged fill deposits, are common throughout the valley and often overlie confined or semi-confined groundwater. When they occur near the land surface, the silt and clay lenses can also support thin perched aquifers that offer a degree of separation between the shallowest layer of groundwater and the more extensive groundwater system below. The low-permeability lenses, although common, seldom are laterally extensive and are often reported as discontinuous or patchy. Regional groundwater flow is from the valley uplands to the valley floor and the Duwamish River.
As groundwater moves from recharge areas to discharge areas, it generally has an upward vertical component as it approaches the discharge zone—that is, the Duwamish River. The typical upward gradient of groundwater flow at discharge zones is further expected in the lower Duwamish River valley because of the presence of the salt wedge in the Duwamish River, which, in addition to its longitudinal extent up the river, extends laterally into the nearshore terrestrial environment. Owing to its greater density, salt water sinks into the ground and underlies the riverbed in the lower reaches of the Duwamish River—where the salt wedge is present—and migrates into the nearshore sediments. At the contact between the salt wedge and the fresh groundwater in the aquifer, some degree of mixing and diffusion occurs, creating a transition zone between the two endmembers. As fresh groundwater flows toward the river, the density contrast between the fresh groundwater and the salty groundwater forces most of the fresh groundwater to flow above the mixing zone and salt wedge and discharge at the shoreline. The large tidal range of the Duwamish River—averaging about 11.4 ft and sometimes exceeding 16 ft—introduces further complexity into the groundwater-discharge regime. At high tide, the river stage exceeds the nearshore groundwater level; thus, the typical flow gradient reverses and river water and the nearshore groundwater flow inland. The net movement of groundwater remains toward the river over each tidal cycle.
Groundwater and surface-water interactions in the lower Duwamish Waterway are inherently complex as a result of three overarching factors. First, water levels in the lower reaches of the Duwamish River vary daily by 11–16 ft because of tides from Puget Sound, which create large swings in the hydraulic gradient in the nearshore groundwater system. Second, the density and chemical composition of water in the Duwamish River change daily with the tides and seasonally, which constrains how river water entering the nearshore sediments interacts with discharging groundwater. Third, the nearshore subsurface and shoreline conditions are heterogenous because of extensive shoreline armoring over the past century, which governs the flow of groundwater and infiltrating river water. These unique features of groundwater and surface-water interactions in the lower Duwamish Waterway thus govern the transport of terrestrial contaminants to the lower Duwamish Waterway. Furthermore, the heterogenous aquifer properties in the lower Duwamish Waterway contribute to spatially and temporally dynamic contaminant-transport processes.
The current study identified several data gaps and uncertainties regarding groundwater and surface-water interactions and implications for contaminant transport in the lower Duwamish Waterway. Groundwater-level and salinity data from groundwater wells—including relatively deep wells, for which data currently are uncommon—close to the river would help in providing an understanding of the extent of the terrestrial part of the salt wedge and the advective processes controlling flow. Long-term data would provide information about how extreme tidal, river-discharge, and precipitation events affect conditions in the nearshore mixing zone. A comprehensive survey to determine the extent of the shallow confining unit in the valley would help characterize how terrestrial contaminants would move toward the lower Duwamish Waterway. Additional data describing contaminant concentrations in pore water and seeps are needed to understand the contaminant fluxes to the lower Duwamish Waterway from the adjacent groundwater system. Groundwater and surface-water interactions along the lower Duwamish Waterway are complex and more information is needed to characterize contaminant transport and to provide guidance to water-resource managers in the area.
Acknowledgments
The authors would like to acknowledge the U.S. Geological Survey (USGS) colleagues who assisted with data collection, including James Foreman, Cameron Marshall, Jennifer McLean, Chad Opatz, and Craig Senter. Additional thanks are given to the staff from the U.S. Geological Survey Washington Water Science Center who supported the project by assisting with administration, data management, and technical reviews.
References Cited
Anchor, Q.E.A., LLC., 2019, Remedial investigation report public review draft, Duwamish Shipyard, Inc. site, Seattle, Washington: Prepared for Washington Department of Ecology by Anchor, Q.E.A., LLC., Seattle, Washington, on behalf of Duwamish Shipyard, Inc., Seattle, Washington, accessed February 14, 2023, at https://apps.ecology.wa.gov/cleanupsearch/site/54.
Anderson, M.P., 2005, Heat as a ground water tracer: Ground Water, v. 43, no. 6, p. 951–968, accessed December 27, 2023,at https://doi.org/10.1111/j.1745-6584.2005.00052.x.
Ando, M., and Balazs, E.I., 1979, Geodetic evidence for aseismic subduction of the Juan de Fuca Plate: Journal of Geophysical Research, v. 84, no. B6, p. 3023–3028, accessed June 13, 2023, at https://doi.org/10.1029/JB084iB06p03023.
Armstrong, J.E., Crandell, D.R., Easterbrook, D.J., and Noble, J.B., 1965, Late Pleistocene stratigraphy and chronology in southwestern British Columbia and northwestern Washington: Geological Society of America Bulletin, v. 76, no. 3, p. 321–330, accessed June 19, 2023, at https://doi.org/10.1130/0016-7606(1965)76[321:LPSACI]2.0.CO;2.
Bauer, H.H., and Vaccaro, J.J., 1987, Documentation of a deep percolation model for estimating ground-water recharge: U.S. Geological Survey Open-File Report 86–536, 180 p., accessed August 20, 2023, at https://doi.org/10.3133/ofr86536.
Blakely, R.J., Wells, R.E., Weaver, C.S., and Johnson, S.Y., 2002, Location, structure, and seismicity of the Seattle fault zone, Washington—Evidence from aeromagnetic anomalies, geologic mapping, and seismic-reflection data: Geological Society of America Bulletin, v. 114, no. 2, p. 169–177, accessed August 15, 2023, at https://doi.org/10.1130/0016-7606(2002)114%3C0169:LSASOT%3E2.0.CO;2.
Booth, D.B., 1987, Timing and processes of deglaciation along the southern margin of the Cordilleran ice sheet, in Ruddiman, W.F., and Wright, H.E. Jr., eds., North America and adjacent oceans during the last deglaciation: The Geology of North America, K-3, Geological Society of America, Boulder, Colo., p. 71–90, accessed June 13, 2023, at https://doi.org/10.1130/DNAG-GNA-K3.71.
Booth, D.B., 1994, Glaciofluvial infilling and scour of the Puget Lowland, Washington, during ice-sheet glaciation: Geology, v. 22, no. 8, p. 695–698, accessed June 13, 2023, at https://faculty.washington.edu/dbooth/Booth%20GEOLOGY%201994.pdf.
Booth, D.B., Troost, K.G., Clague, J.J., and Waitt, R.B., 2003, The Cordilleran ice sheet in Gillespie, A.R., Porter, S.C., and Atwater, B.F., eds., The Quaternary period in the United States: Developments in Quaternary Sciences, v. 1, p. 17–43, accessed August 17, 2023, at https://doi.org/10.1016/S1571-0866(03)01002-9.
Bucknam, R.C., Hemphill-Haley, E., and Leopold, E.B., 1992, Abrupt uplift within the past 1,700 years at southern Puget Sound, Washington: Science, v. 258, no. 5088, p. 1611–1614, accessed August 15, 2023,at https://doi.org/10.1126/science.258.5088.1611.
Carlson, L., 1950, Duwamish River—Its place in the Seattle industrial plan: Economic Geography, v. 26, no. 2, p. 144–154, accessed August 16, 2023, at https://doi.org/10.2307/141732.
Conn, K.E., Black, R.W., Senter, C.A., Peterson, N.T., and Vanderpool-Kimura, A., 2018, Hydrology-driven chemical loads transported by the Green River to the Lower Duwamish Waterway near Seattle, Washington, 2013–17: U.S. Geological Survey Scientific Investigations Report 2018–5133, 37 p., accessed March 29, 2023, at https://doi.org/10.3133/sir20185133.
Cunningham, W.L., and Schalk, C.W., comps., 2011, Groundwater technical procedures of the U.S. Geological Survey: U.S. Geological Survey Techniques and Methods, 151 p., accessed September 4, 2023, at https://doi.org/10.3133/tm1A1.
Dawson, W.A., and Tilley, L.J., 1972, Measurement of salt-wedge excursion distance in the Duwamish River Estuary, Seattle, Washington, by means of the dissolved-oxygen gradient: U.S. Geological Survey Water Supply Paper 1873–D, 27 p., accessed August 23, 2023, at https://doi.org/10.3133/wsp1873D.
Dewitz, J., and U.S. Geological Survey, 2021, National Land Cover Database (NLCD) 2019 Products (ver. 2.0, June 2021): U.S. Geological Survey data release, accessed March 15, 2023, at https://doi.org/10.5066/P9KZCM54.
Ding, Y., and Peng, Y., 2009, Contaminant transport in coastal aquifers: prepared for New Jersey Department of Environmental Protection, 294 p., accessed September 4, 2023, at https://dspace.njstatelib.org/xmlui/bitstream/handle/10929/23257/p7772009i.pdf?sequence=1.
ERM-West, Inc., 2020, Draft remedial investigation/feasibility study report—Volume 1, 5900 West Marginal Way Site: ERM, Seattle, Washington, prepared for Glacier Northwest, Inc., 107 p., accessed March 20, 2023, at https://apps.ecology.wa.gov/cleanupsearch/site/599#site-documents.
Evans, T.B., and Wilson, A.M., 2016, Groundwater transport and the freshwater-saltwater interface below sandy beaches: Journal of Hydrology, v. 538, p. 563–573, accessed September 5, 2023, at https://doi.org/10.1016/j.jhydrol.2016.04.014.
Fischer, H.B., Stoner, J.D., Haushild, W.L., and McConnell, J.B., 1975, A numerical model of material transport in salt-wedge estuaries: U.S. Geological Survey Professional Paper 917, 36 p., accessed August 24, 2023, at https://doi.org/10.3133/pp917.
Fritz, B.G., and Arntzen, E.V., 2007, Effect of rapidly changing river stage on uranium flux through the hyporheic zone: Ground Water, v. 45, no. 6, p. 753–760, accessed September 8, 2023, at https://doi.org/10.1111/j.1745-6584.2007.00365.x.
Galster, R.W., and Laprade, W.T., 1991, Geology of Seattle, Washington, United States of America: Environmental & Engineering Geoscience, v. 28, no. 3, p. 235–302, accessed June 15, 2023, at https://doi.org/10.2113/gseegeosci.xxviii.3.235.
GeoEngineers, Inc., 2019, Public review draft final remedial investigation report—Douglas Management Dock: Prepared by GeoEngineers, Inc., Seattle, Washington, for 7100 1st Ave. S. Seattle LLC, Seattle Washington, accessed December 12, 2022, at https://apps.ecology.wa.gov/cleanupsearch/site/6967.
Geng, X., Boufadel, M.C., Lee, K., Abrams, S., and Suidan, M., 2015, Biodegradation of subsurface oil in a tidally influenced sand beach—Impact of hydraulics and interaction with pore water chemistry: Water Resources Research, v. 51, no. 5, p. 3193–3218, accessed September 4, 2023, at https://doi.org/10.1002/2014WR016870.
Grassi, S., and Netti, R., 2000, Sea water intrusion and mercury pollution of some coastal aquifers in the province of Grosseto (Southern Tuscany, Italy): Journal of Hydrology, v. 237, nos. 3–4, p. 198–211, accessed September 4, 2023, at https://doi.org/10.1016/S0022-1694(00)00307-3.
Hahne, H.C.H., and Kroontje, W., 1973, Significance of pH and chloride concentration on behavior of heavy metal pollutants—Mercury(II), cadmium (II), zinc (II), and lead (II): Journal of Environmental Quality, v. 2, no. 4, p. 444–450, accessed September 5, 2023, at https://doi.org/10.2134/jeq1973.00472425000200040007x.
Haugerud, R.A., Troost, K.G., and Laprade, W.T., 2017, Geology of Seattle, a field trip in Haugerud, R.A., and Kelsey, H.M., eds., From the Puget Lowland to east of the Cascade Range—Geologic excursions in the Pacific Northwest: Geological Society of America, GSA Field Guides, v. 49, 24 p., accessed August 17, 2023, at https://doi.org/10.1130/2017.0049(01).
Heerhartz, S.M., Dethier, M.N., Toft, J.D., Cordell, J.R., and Ogston, A.S., 2014, Effects of shoreline armoring on beach wrack subsidies to the nearshore ecotone in an estuarine fjord: Estuaries and Coasts, v. 37, no. 5, p. 1256–1268, accessed August 24, 2023, at https://doi.org/10.1007/s12237-013-9754-5.
In-Situ Inc., 2023, Aqua TROLL CTD data loggers: In-Situ Inc. website, accessed August 25, 2023, at https://in-situ.com/pub/media/support/documents/aquatroll100-200_spec-sheet_ltr_en.pdf.
Jones, M.A., 1996, Thickness of unconsolidated deposits in the Puget Sound Lowland, Washington and British Columbia: U.S. Geological Survey Water-Resources Investigations Report 94–4133, accessed June 20, 2023, at https://doi.org/10.3133/wri944133.
King County, 2013, 2012 high-resolution natural color orthoimagery for the Puget Sound region (WA) NGA urban area: Acquisition date March 23, 2012, accessed December 4, 2023, at https://earthexplorer.usgs.gov/.
King County, 2018, Lower Duwamish waterway source control—Green River watershed surface water data report: Prepared for King County Wastewater Treatment Division, by Greyell, C., Williston, D., and Lester, D., 84 p., accessed September 8, 2023, at https://kingcounty.gov/en/dept/dnrp/waste-services/wastewater-treatment/programs/duwamish-waterway/lower-duwamish/library-and-resources.
La Licata, I., Langevin, C.D., and Dausman, A.M., 2007, Effect of tidal fluctuations on contaminant transfer to the ocean: Proceedings of the International Symposium—A New Focus on Groundwater—Seawater Interactions, Perugia, Italy, July 2007, p. 334–340, accessed September 1, 2023, at https://iahs.info/uploads/dms/13959.46-334-342-312-13_LaLicata.pdf.
Leidos, Inc., 2017, Lower Duwamish Waterway groundwater sampling for PCB congeners and aroclors—Data report: Prepared for the Washington State Department of Ecology, by Leidos, Inc., Bothell, Washington, 466 p., accessed September 8, 2023, at https://apps.ecology.wa.gov/cleanupsearch/site/1643#site-documents.
Li, L., Barry, D.A., Jeng, D.-S., and Prommer, H., 2003, Tidal dynamics of groundwater flow and contaminant transport in coastal aquifers, chap. 6 of Cheng, A.H.D., and Ouazar, D., eds., Coastal aquifer management—Monitoring, modeling, and case studies: Boca Raton, Florida, CRC Press, p. 115–141, accessed September 8, 2023, at https://www.researchgate.net/publication/37426949_Tidal_Dynamics_of_Groundwater_Flow_and_Contaminant_Transport_in_Coastal_Aquifers.
Li, C., Zhang, C., Gibbes, B., Wang, T., and Lockington, D., 2022, Coupling effects of tide and salting-out on perfluorooctane sulfonate (PFOS) transport and adsorption in a coastal aquifer: Advances in Water Resources, v. 166, 11 p., accessed August 1, 2023, at https://doi.org/10.1016/j.advwatres.2022.104240.
Li, L., Barry, D.A., Stagnitti, F., and Parlange, J.-Y., 1999, Submarine groundwater discharge and associated chemical input to a coastal sea: Water Resources Research, v. 35, no. 11, p. 3253–3259, accessed September 4, 2023, at https://doi.org/10.1029/1999WR900189.
Lower Duwamish Waterway Group, 2004, Lower Duwamish Waterway bathymetric survey: Portland, Oregon, David Evans and Associates, Inc., prepared for Windward Environmental, LLC., accessed June 29, 2023, at https://ldwg.org/wp-content/uploads/2019/01/Bathymetry_report.pdf.
Lower Duwamish Waterway Group, 2010, Lower Duwamish Waterway remedial investigation report: Prepared by Windward Environmental, LLC., Seattle, Washington, for Lower Duwamish Water Group for submission to U.S Environmental Protection Agency and Washington State Department of Ecology, 826 p., accessed February 10, 2023, at https://ldwg.org/wp-content/uploads/2018/12/Final_LDW_RI.pdf.
Mastin, M.C., Konrad, C.P., Veilleux, A.G., and Tecca, A.E., 2016, Magnitude, frequency, and trends of floods at gaged and ungaged sites in Washington, based on data through water year 2014 (ver. 1.2, November 2017): U.S. Geological Survey Scientific Investigations Report 2016–5118, 70 p., accessed August 22, 2023, at https://doi.org/10.3133/sir20165118.
McKeon, M.A., Horner-Devine, A.R., and Giddings, S.N., 2020, Seasonal changes in structure and dynamics in an urbanized salt wedge estuary: Estuaries and Coasts, v. 44, p. 589–607, accessed September 8, 2023, at https://doi.org/10.1007/s12237-020-00788-z.
Millward, G.E., and Liu, Y.P., 2003, Modelling metal desorption kinetics in estuaries: Science of the Total Environment, v. 314–316, p. 613–623, accessed August 15, 2023, at https://doi.org/10.1016/S0048-9697(03)00077-9.
Minard, J.P., and Booth, D.B., 1988, Geologic map of the Redmond Quadrangle, King County, Washington: U.S. Geological Survey Miscellaneous Field Studies Map MF–2016, scale 1:24,000, accessed August 18, 2023, at https://doi.org/10.3133/mf2016.
Mitchell, J.N., and Opatz, C.C., 2024, Data in support of groundwater- and surface-water-interactions report—Groundwater and surface-water interactions in the Lower Duwamish Waterway, Seattle, Washington: U.S. Geological Survey data release, https://doi.org/10.5066/P1F7QX42.
Moore, W.S., 1999, The subterranean estuary—A reaction zone of groundwater and sea water: Marine Chemistry, v. 65, nos. 1–2, p. 111–125, accessed July 17, 2023, at https://doi.org/10.1016/S0304-4203(99)00014-6.
Morley, S.A., Toft, J.D., and Hanson, K.M., 2012, Ecological effects of shoreline armoring on intertidal habitats of a Puget Sound urban estuary: Estuaries and Coasts, v. 35, no. 3, p. 774–784, accessed June 22, 2023, at https://doi.org/10.1007/s12237-012-9481-3.
Mullineaux, D.R., 1970, Geology of the Renton, Auburn, and Black Diamond quadrangles, King County, Washington: U.S. Geological Survey Professional Paper 672, 92 p., accessed June 15, 2023, at https://doi.org/10.3133/pp672.
Mullineaux, D.R., Waldron, H.H., and Rubin, M., 1965, Stratigraphy and chronology of late interglacial and early Vashon glacial time in the Seattle area, Washington: U.S. Geological Survey Bulletin 1194-O, 10 p., accessed June 13, 2023, at https://doi.org/10.3133/b1194O.
National Oceanic and Atmospheric Administration, 2023a, Climate data online: NOAA National Centers for Environmental Information, accessed August 23, 2023, at https://www.ncdc.noaa.gov/cdo-web/.
National Oceanic and Atmospheric Administration, 2023b, Tides & currents: NOAA Tides and Water Levels, accessed August 23, 2023, at https://tidesandcurrents.noaa.gov/stations.html?type=Datums.
Parsons, D.R., Jackson, P.R., Czuba, J.A., Engel, F.L., Rhoads, B.L., Oberg, K.A., Best, J.L., Mueller, D.S., Johnson, K.K., and Riley, J.D., 2012, Velocity mapping toolbox (VMT)—A processing and visualization suite for moving-vessel ADCP measurements: Earth Surface Processes and Landforms, v. 38, no. 11, p. 1244–1260, accessed September 1, 2023, at https://doi.org/10.1002/esp.3367.
Polizzotto, M.L., Kocar, B.D., Benner, S.G., Sampson, M., and Fendorf, S., 2008, Near-surface wetland sediments as a source of arsenic release to ground water in Asia: Nature, v. 454, no. 7203, p. 505–508, accessed September 11, 2023, at https://doi.org/10.1038/nature07093.
Porter, S.C., and Swanson, T.W., 1998, Radiocarbon age constraints on rates of advance and retreat of the Puget Lobe of the Cordilleran ice sheet during the last glaciation: Quaternary Research, v. 50, no. 3, p. 205–213, accessed June 19, 2023, at https://doi.org/10.1006/qres.1998.2004.
Robinson, C., Brovelli, A., Barry, D.A., and Li, L., 2009, Tidal influence on BTEX biodegradation in sandy coastal aquifers: Advances in Water Resources, v. 32, no. 1, p. 16–28, accessed September 12, 2023, at https://doi.org/10.1016/j.advwatres.2008.09.008.
Robinson, C., Li, L., and Barry, D.A., 2007, Effect of tidal forcing on a subterranean estuary: Advances in Water Resources, v. 30, no. 4, p. 851–865, accessed September 12, 2023, at https://doi.org/10.1016/j.advwatres.2006.07.006.
Robinson, C.E., Xin, P., Santos, I.R., Charette, M.A., Li, L., and Barry, D.A., 2018, Groundwater dynamics in subterranean estuaries of coastal unconfined aquifers—Controls on submarine groundwater discharge and chemical inputs to the ocean: Advances in Water Resources, v. 115, p. 315–331, accessed September 8, 2023, at https://doi.org/10.1016/j.advwatres.2017.10.041.
Rosenberry, D.O., Sheibley, R.W., Cox, S.E., Simonds, F.W., and Naftz, D.L., 2013, Temporal variability of exchange between groundwater and surface water based on high-frequency direct measurements of seepage at the sediment-water interface: Water Resources Research, v. 49, no. 5, p. 2975–2986, accessed July 7, 2023, at https://doi.org/10.1002/wrcr.20198.
SAIC and Newfields, 2011, Stormwater lateral loading study, Lower Duwamish Waterway, WA: Data report, prepared for the Washington State Department of Ecology by SAIC, Bothell, Washington, and NewFields, Edmonds, Washington, 48 p., accessed September 8, 2023, at https://apps.ecology.wa.gov/cleanupsearch/site/1643#site-documents.
Santos, J.F., and Stoner, J.D., 1972, Physical, chemical, and biological aspects of the Duwamish River Estuary, King County, Washington, 1963–67: U.S. Geological Survey Water Supply Paper 1873, 74 p., accessed August 22, 2023, at https://doi.org/10.3133/wsp1873C.
Senter, C.A., Conn, K.E., Black, R.W., Peterson, N., Vanderpool-Kimura, A., and Foreman, J.R., 2018, Suspended-sediment transport from the Green-Duwamish River to the Lower Duwamish Waterway, Seattle, Washington, 2013–17: U.S. Geological Survey Open-File Report 2018–1029, 23 p., accessed September 8, 2023, at https://doi.org/10.3133/ofr20181029.
Senter, C.A., Conn, K.E., Black, R.W., Welch, W.B., and Fasser, E.T., 2019, Assessment of existing groundwater quality data in the Green-Duwamish watershed, Washington: U.S. Geological Survey Open-File Report 2019–1131, 35 p., accessed September 8, 2023, at https://doi.org/10.3133/ofr20191131.
Serfes, M.E., 1991, Determining the mean hydraulic gradient of ground water affected by tidal fluctuations: Ground Water, v. 29, no. 4, p. 549–555, accessed March 17, 2023, at https://doi.org/10.1111/j.1745-6584.1991.tb00546.x.
Simenstad, C., Ramirez, M., Burke, J., Logsdon, M., Shipman, H., Tanner, C., Toft, J., Craig, B., Davis, C., Fung, J., Bloch, P., Fresh, K., Campbell, S., Myers, D., Iverson, E., Bailey, A., Schlenger, P., Kiblinger, C., Myre, P., Gertsel, W.I., and MacLennan, A., 2011, Historical change and impairment of Puget Sound shorelines: Olympia, Washington, Washington Department of Fish and Wildlife, and Seattle, Washington, U.S. Army Corps of Engineers, Puget Sound Nearshore Report Number 2011-01, 289 p., accessed August 24, 2023, at https://wdfw.wa.gov/sites/default/files/publications/02186/wdfw02186.pdf.
Simenstad, C., Tanner, C., Crandell, C., White, J., and Cordell, J., 2005, Challenges of habitat restoration in a heavily urbanized estuary—Evaluating the investment: Journal of Coastal Research, Special Issue no. 40, p. 6–23, accessed August 23, 2023, at https://www.jstor.org/stable/25736612.
Simonds, F.W., Swarzenski, P.W., Rosenberry, D.O., Reich, C.D., and Paulson, A.J., 2008, Estimates of nutrient loading by ground-water discharge into the Lynch Cover area of Hood Canal, Washington: U.S. Geological Survey Scientific Investigations Report 2008–5078, 55 p., accessed September 7, 2023, at https://doi.org/10.3133/sir20085078.
Smith, K.A., O’Sullivan, A.M., Kennedy, G., Benz, S.A., Somers, L.D., and Kurylyk, B.L., 2023, Shallow groundwater temperature patterns revealed through a regional monitoring well network: Hydrological Processes, v. 37, no. 9, 14 p., accessed December 27, 2023, at https://doi.org/10.1002/hyp.14975.
Stoner, J.D., 1967, Prediction of salt-water intrusion in the Duwamish River estuary, King County, Washington, in Geological Survey research—1967: U.S. Geological Survey Professional Paper 575–D, p. D253–D255, accessed August 23, 2023, at https://doi.org/10.3133/pp575D.
Thorson, R.M., 1980, Ice-sheet glaciation of the Puget Lowland, Washington, during the Vashon stade (late Pleistocene): Quaternary Research, v. 13, no. 3, p. 303–321, accessed August 17, 2023, at https://doi.org/10.1016/0033-5894(80)90059-9.
Tiffreau, C., Lützenkirchen, J., and Behra, P., 1995, Modeling the adsorption of mercury(II) on (hydr)oxides—I. amorphous iron oxide and α-quartz: Journal of Colloid and Interface Science, v. 172, no. 1, p. 82–93, accessed September 4, 2023, at https://doi.org/10.1006/jcis.1995.1228.
Troost, K.G., and Booth, D.B., 2008, Geology of Seattle and the Seattle area, Washington, in Baum, R.L, Godt, J.W., and Highland, L.M., eds., Landslides and engineering geology of the Seattle, Washington, area: Geological Society of America Reviews in Engineering Geology, v. 20, p. 1–35, accessed June 13, 2023, at https://doi.org/10.1130/2008.4020(01).
Troost, K.G., Booth, D.B., Wisher, A.P., and Shimel, S.A., 2005, The geologic map of Seattle—A progress report: U.S. Geological Survey Open-File Report 2005–1252, 1 pl., accessed December 1, 2022, at https://doi.org/10.3133/ofr20051252.
U.S. Environmental Protection Agency, 2014, Record of decision—Lower Duwamish Waterway Superfund Site: U.S. Environmental Protection Agency Region 10, accessed August 1, 2023, at https://semspub.epa.gov/work/10/715975.pdf.
U.S. Geological Survey, 1894, Seattle, Washington: U.S. Geological Survey 15 × 15 minute topographic quadrangle, scale 1:62,500, accessed March 29, 2023, at https://ngmdb.usgs.gov/topoview/.
U.S. Geological Survey, 2023a, 3D Elevation Program 1-meter resolution digital elevation model: U.S. Geological Survey database accessed February 3, 2023, at https://www.usgs.gov/the-national-map-data-delivery.
U.S. Geological Survey, 2023b, StreamStats: U.S. Geological Survey website, accessed August 22, 2023, at https://streamstats.usgs.gov/ss/.
U.S. Geological Survey, 2023c, USGS water data for the Nation: U.S. Geological Survey National Water Information System website, accessed August 23, 2023, at https://doi.org/10.5066/F7P55KJN.
Vine, J.D., 1962, Stratigraphy of Eocene rocks in a part of King County, Washington: Washington Division of Mines and Geology Report of Investigation 21, 20 p., accessed June 13, 2023, at https://file.dnr.wa.gov/publications/ger_ri21_strat_eocene_king_co.pdf.
Wagner, R.J., Boulger, R.W., Jr., Oblinger, C.J., and Smith, B.A., 2006, Guidelines and standard procedures for continuous water-quality monitors—Station operation, record computation, and data reporting: U.S. Geological Survey Techniques and Methods book 1, chap. D3, 51 p., accessed September 4, 2023, at https://doi.org/10.3133/tm1D3.
Washington State Department of Ecology, 2023f, Cleanup and Tank Search: Washington State Department of Ecology cleanup and tank search website, accessed September 4, 2023, at https://apps.ecology.wa.gov/cleanupsearch/.
Washington State Department of Ecology, 2023d, Environmental Information Management System: Washington State Department of Ecology EIM website, accessed September 4, 2023, at https://apps.ecology.wa.gov/eim/search/default.aspx.
Washington State Department of Ecology, 2023b, Glacier Northwest Inc.: Washington State Department of Ecology Cleanup and Tank Search website, accessed September 4, 2023, at https://apps.ecology.wa.gov/cleanupsearch/site/599#site-documents.
Washington State Department of Ecology, 2023a, Lower Duwamish Waterway: Washington State Department of Ecology cleanup sites website, accessed September 4, 2023, at https://ecology.wa.gov/spills-cleanup/contamination-cleanup/cleanup-sites/lower-duwamish-waterway.
Washington State Department of Ecology, 2023c, Source control: Washington State Department of Ecology source control website, accessed September 4, 2023, at https://ecology.wa.gov/spills-cleanup/contamination-cleanup/cleanup-sites/lower-duwamish-waterway/source-control.
Washington State Department of Natural Resources, 2023, Geology GIS data and databases: Washington State Department of Natural Resources geology GIS data and databases website, accessed September 4, 2023, at https://www.dnr.wa.gov/programs-and-services/geology/publications-and-data/gis-data-and-databases.
Washington State Legislature, 1989, Hazardous waste cleanup—Model Toxics Control Act: Revised Code of Washington, chap. 70A.305, accessed August 23, 2023, at https://app.leg.wa.gov/RCW/default.aspx?cite=70A.305.
Weaver, C.E., 1916, The Tertiary formations of western Washington: Washington Geological Survey Bulletin, no. 13, 327 p., accessed June 13, 2023, at https://www.dnr.wa.gov/publications/ger_b13_teriary_form_western_wa_1.pdf.
Westbrook, S.J., Rayner, J.L., Davis, G.B., Clement, T.P., Bjerg, P.L., and Fisher, S.J., 2005, Interaction between shallow groundwater, saline surface water and contaminant discharge at a seasonally and tidally forced estuarine boundary: Journal of Hydrology, v. 302, nos. 1–4, p. 255–269, accessed August 1, 2023, at https://doi.org/10.1016/j.jhydrol.2004.07.007.
Wong, V.N.L., Johnston, S.G., Burton, E.D., Bush, R.T., Sullivan, L.A., and Slavich, P.G., 2010, Seawater causes rapid trace metal mobilization in coastal lowland acid sulfate soils—Implications of sea level rise for water quality: Geoderma, v. 160, no. 2, p. 252–263, accessed September 8, 2023, at https://doi.org/10.1016/j.geoderma.2010.10.002.
Woodward, D.G., Packard, F.A., Dion, N.P, and Sumioka, S.S., 1995, Occurrence and quality of ground water in southwestern King County, Washington: U.S. Geological Survey Water-Resources Investigations Report 92–4098, 69 p., accessed September 8, 2023, at https://doi.org/10.3133/wri924098.
Yager, R.M., Welch, W.B., Headman, A., and Dinicola, R.S., 2020, Variable-density groundwater flow and contaminant transport, Operable Unit 1, Naval Base Kitsap, Keyport, Washington: U.S. Geological Survey Scientific Investigations Report 2020–5066, 58 p., accessed August 3, 2023, at https://doi.org/10.3133/sir20205066.
Yim, C.S., and Mohsen, M.F.N., 1992, Simulation of tidal effects on contaminant transport in porous media: Ground Water, v. 30, no. 1, p. 78–86, accessed September 12, 2023, https://doi.org/10.1111/j.1745-6584.1992.tb00814.x.
Yount, J.C., Dembroff, G.R., and Barats, G.M., 1985, Map showing depth to bedrock in the Seattle 30′ by 60′ quadrangle—Washington: U.S. Geological Survey Miscellaneous Field Studies Map 1692, accessed August 18, 2023, https://doi.org/10.3133/mf1692.
Yount, J.C., and Gower, H.D., 1991, Bedrock geologic map of Seattle 30' by 60' quadrangle, Washington: U.S. Geological Survey Open-File Report 91–147, 37 p., 4 pls., accessed August 18, 2023, at https://doi.org/10.3133/ofr91147.
Yu, X., LeMonte, J.J., Li, J., Stuckey, J.W., Sparks, D.L., Cargill, J.G., Russoniello, C.J., and Michael, H.A., 2023, Hydrologic control on arsenic cycling at the groundwater-surface water interface of a tidal channel: Environmental Science & Technology, v. 57, no. 1, p. 222–230, accessed September 12, 2023, at https://doi.org/10.1021/acs.est.2c05930.
Conversion Factors
U.S. customary units to International System of Units
Multiply | By | To obtain |
---|---|---|
inch (in.) | 2.54 | centimeter (cm) |
foot (Suggest parenthetically adding legal citations for each of these two laws.) | 0.3048 | meter (m) |
mile (mi) | 1.609 | kilometer (km) |
acre | 4,047 | square meter (m2) |
square mile (mi2) | 2.590 | square kilometer (km2) |
cubic foot (ft3) | 0.02832 | cubic meter (m3) |
foot per day (ft/d) | 0.3048 | meter per day (m/d) |
International System of Units to U.S. customary units
Temperature in degrees Celsius (°C) may be converted to degrees Fahrenheit (°F) as follows:
°F = (1.8 × °C) + 32.
Temperature in degrees Fahrenheit (°F) may be converted to degrees Celsius (°C) as follows:
°C = (°F – 32) / 1.8.
Datums
Vertical coordinate information is referenced to the North American Vertical Datum of 1988 (NAVD 88).
Horizontal coordinate information is referenced to the North American Datum of 1983 (NAD 83).
Altitude, as used in this report, refers to distance above the vertical datum (where known).
Supplemental Information
Specific conductance is given in microsiemens per centimeter at 25 degrees Celsius (µS/cm at 25 °C).
Concentrations of chemical constituents in water are given in either milligrams per liter (mg/L) or micrograms per liter (µg/L).
Abbreviations
ADCP
acoustic Doppler current profiler
ka
thousand years ago
MHHW
mean higher high water
MLLW
mean lower low water
NAVD 88
North American Vertical Datum of 1988
PAHs
polycyclic aromatic hydrocarbons
PCBs
polychlorinated biphenyls
PFOS
perfluorooctane sulfonate
RI/FS
remedial investigation and feasibility study
RM
river mile
USGS
U.S. Geological Survey
For information about the research in this report, contact the
Director, Washington Water Science Center
U.S. Geological Survey
934 Broadway, Suite 300
Tacoma, Washington 98402
https://www.usgs.gov/centers/washington-water-science-center
Manuscript approved on May 24, 2024
Publishing support provided by the U.S. Geological Survey
Science Publishing Network, Tacoma Publishing Service Center
Edited by Vanessa Ball and John Osias
Layout and design by Luis Menoyo
Disclaimers
Any use of trade, firm, or product names is for descriptive purposes only and does not imply endorsement by the U.S. Government.
Although this information product, for the most part, is in the public domain, it also may contain copyrighted materials as noted in the text. Permission to reproduce copyrighted items must be secured from the copyright owner.
Suggested Citation
Mitchell, J.N., and Conn, K.E., 2024, Groundwater and surface-water interactions in the Lower Duwamish Waterway, Seattle, Washington: U.S. Geological Survey Scientific Investigations Report 2024–5046, 43 p., https://doi.org/10.3133/sir20245046.
ISSN: 2328-0328 (online)
Study Area
Publication type | Report |
---|---|
Publication Subtype | USGS Numbered Series |
Title | Groundwater and surface-water interactions in the Lower Duwamish Waterway, Seattle, Washington |
Series title | Scientific Investigations Report |
Series number | 2024-5046 |
DOI | 10.3133/sir20245046 |
Year Published | 2024 |
Language | English |
Publisher | U.S. Geological Survey |
Publisher location | Reston, VA |
Contributing office(s) | Washington Water Science Center |
Description | Report: vi, 43 p.; Data Release |
Country | United States |
State | Washington |
City | Seattle |
Other Geospatial | Lower Duwamish Waterway |
Online Only (Y/N) | Y |