Potential Effects of Climate Change on Acipenser fulvescens (Lake Sturgeon)
Links
- Document: Report (29 MB pdf) , HTML , XML
- Larger Work: This publication is Chapter E of Effects of climate change on fish and wildlife species in the United States
- Download citation as: RIS | Dublin Core
Acknowledgments
We would like to thank Kim Chanell of Great Lakes Integrated Sciences and Assessments (GLISA) for providing edits and comments on this report. We would like to thank Craig Paukert of the U.S. Geological Survey for providing edits and comments on this report. This research was funded by the U.S. Geological Survey Midwest Climate Adaptation Science Center and the Southeast Climate Adaptation Science Center. This research also was supported in part by an appointment to the U.S. Geological Survey Research Participation Program administered by the Oak Ridge Institute for Science and Education through an interagency agreement between the U.S. Department of Energy and the U.S. Department of the Interior. The Oak Ridge Institute for Science and Education is managed by Oak Ridge Associated Universities under the U.S. Department of Energy.
Abstract
Acipenser fulvescens (Rafinesque, 1817; lake sturgeon) are the only sturgeon species native to the Great Lakes region and are threatened across most of their range. They are historically vulnerable because of overfishing and habitat fragmentation with the potential for climate change acting as an increasing stressor in the future. Lake sturgeon span multiple habitats during their long lifespans, including high gradient streams, nearshore areas, and deep rivers and lakes. Climate change is projected to strongly affect the suitability of these habitats through increasing precipitation and temperatures and decreasing ice cover and snowmelt. Changes in flow timing and amount can affect movement to spawning and nursery sites, and increased water temperatures are likely to affect species activity patterns, survival, and prey availability. Ultimately, the Great Lakes region is expected to face wide ranging effects from climate change, which may have positive and negative effects on lake sturgeon depending on a variety of factors, including the life stages affected, habitat availability, and interactions with other stressors, but all shifts are likely to affect spawning. Importantly, several areas of further research would be beneficial to understanding the complex effects of climate change on lake sturgeon.
Introduction
Acipenser fulvescens (Rafinesque, 1817; lake sturgeon) have a wide geographical range (fig. 1), and the largest lake sturgeon populations are in the St. Lawrence River east of Montreal, Canada; Lake of the Woods/Rainy River, United States; Lake Winnebago system, United States; and Lake St. Clair, Canada/United States; however, other recovering and remnant populations exist (Kampa and others, 2014; Bruch and others, 2016; Schloesser and Quinlan, 2019; GLIFWC Climate Change Team, 2023). For example, a lake sturgeon population is recovering in the St. Louis River, United States (Stults and others, 2016). Currently, 2 Canadian Provinces have commercial fisheries, whereas 3 U.S. States and 5 Canadian Provinces have recreational fisheries, and 2 U.S. States (Michigan and Wisconsin) and 5 Canadian Provinces support indigenous fisheries (Bruch and others, 2016). Harvest, where practiced, is highly regulated, resulting in predominantly legal harvest.
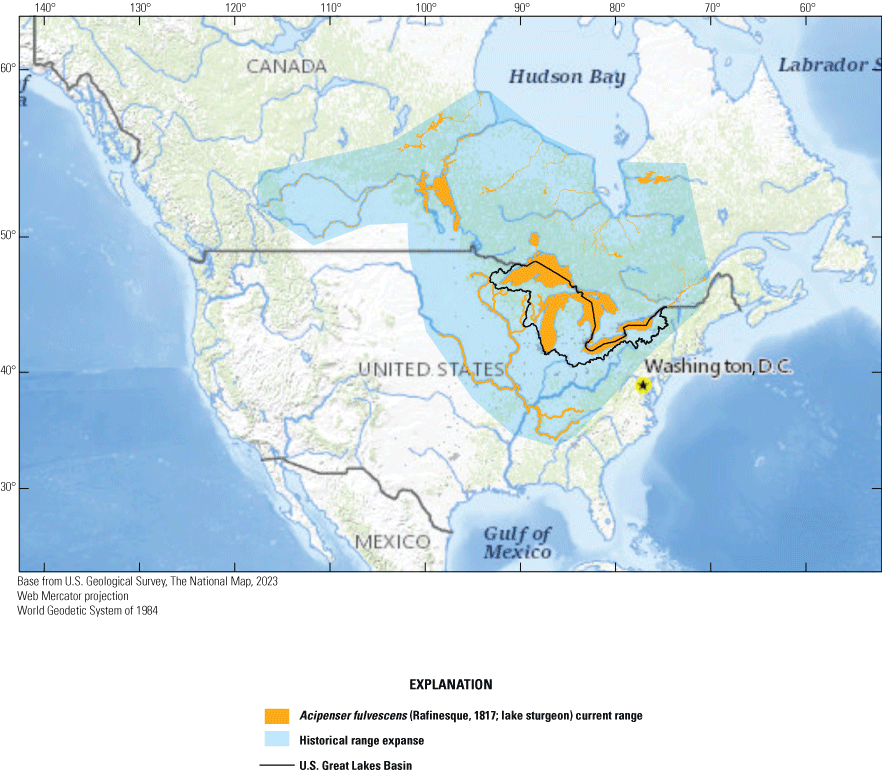
Acipenser fulvescens (Rafinesque, 1817; lake sturgeon) current range, historical range expanse, and U.S. Great Lakes Basin (adapted from the Committee on the Status of Endangered Wildlife in Canada [2006] and NatureServe [2022]).
Lake sturgeon are strongly tied to multiple habitats used during different life stages. Lake sturgeon make large (greater than [>] 200 kilometers [km]) spawning migrations to fast-moving streams in the spring; however, these fish spend a brief part of their lifetime spawning given they are late maturing and intermittently reproduce (Welsh and others, 2017). Most of their time is spent in nearshore lakes and rivers, which provide critical habitat for larvae and juveniles, or in deeper lakes and rivers where adults predominantly spend their nonspawning time. These different life stages are dependent on suitable habitat conditions, which are likely to be affected by climate (fig. 2).
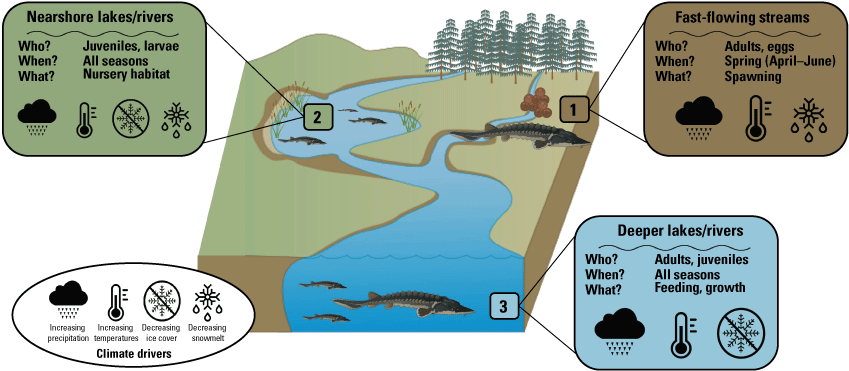
Summary of Acipenser fulvescens (Rafinesque, 1817; lake sturgeon) habitat use and climate drivers.
Lake sturgeon have great ecological, subsistence, and cultural value in the Great Lakes region (Stults and others, 2016). Given the expansive movements lake sturgeon make throughout their long lifetimes, these fish connect habitats and food webs across a wide spatial extent. Additionally, for some indigenous nations including the Menominee Indian Tribe of Wisconsin and the Little River Band of Ottawa Indians, lake sturgeon are a foundational component of indigenous history and considered a close relative responsible for supporting food sovereignty (GLIFWC Climate Change Team, 2023). For recreational anglers, lake sturgeon support a highly popular ice fishery in some areas (for example, the Lake Winnebago system). However, climate change is expected to disproportionately affect many of these relations among lake sturgeon, indigenous communities, and anglers because of geographically limited boundaries of reservations and ceded territories, which may limit communities’ ability to follow species’ shifts (Stults and others, 2016).
The purpose of this report is to provide an overview, based on peer-reviewed literature, government reports, and analysis of regional climate data where appropriate, of the direct and indirect effects of climate change on lake sturgeon. This report is structured around lake sturgeon life stages, habitat needs, and associated stressors. Because of the wide geographic range and mobility of the species, we limit the analysis to a broad consideration of climate effects in the U.S. Great Lakes Basin because this region supports the largest native lake sturgeon populations (fig. 1). We do not provide specific information on Lake St. Clair given limited data despite its importance to lake sturgeon; however, these trends are somewhat captured in the Lake Huron and Lake Erie information.
Data and Methods
To explore the potential range of future climate changes, we used downscaled projections for this region based on the Intergovernmental Panel on Climate Change Coupled Model Intercomparison Project Phase 5 models for two emissions scenarios: a moderate emissions scenario (representative concentration pathway 4.5 [RCP4.5]) and a high emissions scenario (representative concentration pathway 8.5 [RCP8.5]) (Taylor and others, 2012). The output from 20 global climate models with the necessary variables is statistically downscaled using the Multivariate Adaptive Constructed Analogs version 2 (MACAv2) method; this multistep constructed analog approach establishes relations between global climate model output and historical climate observations. Outputs are bias corrected by climate variable to develop higher resolution and localized projections with about a 4-km spatial resolution. The first realization (r1i1p1) of each model is used, except for model CCSM4, which uses the sixth realization (r6i1p1). Output can be accessed through https://climate.northwestknowledge.net/MACA/data_portal.php (Abatzoglou and Brown, 2012), and the full list of models used is provided in table 1. MACAv2 was chosen because it is widely vetted and used for impact studies, is available at the appropriate spatial and temporal resolution for the region of interest, and has been documented to better capture signals in rainfall extremes and frequency compared to other downscaled products (Wang and others, 2020; Wootten and others, 2021). Although the distribution of lake sturgeon extends into Canada, data analysis in this report focuses on climate changes and effects in the United States.
Table 1.
Downscaled global climate models from Multivariate Adaptive Constructed Analogs version 2-METDATA used in this study (Abatzoglou and Brown, 2012).Letter1 | Model | Group | Citation |
---|---|---|---|
A | BNU–ESM | College of Global Change and Earth System Science, Beijing Normal University (GCESS) | Ji and others (2014) |
B | CCSM4 | National Center for Atmospheric Research (NCAR) | Gent and others (2011) |
C | CNRM–CM5 | Centre National de Recherches Meteorologiques/Centre Européen de Recherche et Formation Avances en Calcul Scientifique (CNRM–CERFACS) | Voldoire and others (2013) |
D | CSIRO–Mk3–6–0 | Commonwealth Scientific and Industrial Research Organisation in collaboration with the Queensland Climate Change Centre of Excellence (CSIRO–QCCCE) | Rotstayn and others (2010) |
E | CanESM2 | Canadian Centre for Climate Modeling and Analysis (CCCma) | Arora and others (2011) |
F | GFDL–ESM2G | Geophysical Fluid Dynamics Laboratory (NOAA–GFDL) | Dunne and others (2012) |
G | GFDL–ESM2M | Geophysical Fluid Dynamics Laboratory (NOAA–GFDL) | Dunne and others (2012) |
H | HadGEM2–CC365 | Met Office Hadley Centre (MOHC) | Collins and others (2011) |
I | HadGEM2–ES365 | Met Office Hadley Centre (additional HadGEM2–ES realizations contributed by Instituto Nacional de Pesquisas Espaciais) (MOHC) | Collins and others (2011) |
J | IPSL–CM5A–LR | Institut Pierre-Simon Laplace (IPSL) | Marti and others (2010) |
K | IPSL–CM5A–MR | Institut Pierre-Simon Laplace (IPSL) | Marti and others (2010) |
L | IPSL–CM5B–LR | Institut Pierre-Simon Laplace (IPSL) | Marti and others (2010) |
M | MIROC–ESM–CHEM | Japan Agency for Marine-Earth Science and Technology, Atmosphere and Ocean Research Institute (The University of Tokyo), and National Institute for Environmental Studies (MIROC) | Watanabe and others (2010) |
N | MIROC–ESM | Japan Agency for Marine-Earth Science and Technology, Atmosphere and Ocean Research Institute (The University of Tokyo), and National Institute for Environmental Studies (MIROC) | Watanabe and others (2010) |
O | MIROC5 | Atmosphere and Ocean Research Institute (The University of Tokyo), National Institute for Environmental Studies (MIROC), and Japan Agency for Marine-Earth Science and Technology | Watanabe and others (2010) |
P | MRI–CGCM3 | Meteorological Research Institute (MRI) | Yukimoto and others (2012) |
Q | NorESM1–M | Norwegian Climate Centre (NCC) | Bentsen and others (2013) |
R | bcc–csm1–1–m | Beijing Climate Center, China Meteorological Administration (BCC) | Xiao-Ge and others (2013) |
S | bcc–csm1–1 | Beijing Climate Center, China Meteorological Administration (BCC) | Xiao-Ge and others (2013) |
T | inmcm4 | Institute for Numerical Mathematics (INM) | Volodin and others (2010) |
Current climate modeling efforts have challenges in the Great Lakes region. Some current global climate models do not represent the Great Lakes (Mallard and others, 2015; Briley and others, 2021), and higher resolution modeling studies often use simple lake models (Bennington and others, 2014; Mallard and others, 2014; Xiao and others, 2016; Xue and others, 2017; Sharma and others, 2018; Wang and others, 2022). Projections of hydroclimatic variables such as precipitation and evaporation in the basin may be particularly affected by the inclusion of lake models that better capture energy, momentum, and moisture fluxes and feedback, especially during the winter months when low ice cover enhances lake effect precipitation (Notaro and others, 2021, 2022). Analysis in this report uses statistically downscaled projections from the MACAv2 product. The MACAv2 product provides a larger ensemble of projections at a higher resolution than other regional datasets and is used here as a conservative estimate of the full range of potential risk. We do so with an understanding of the potential drawbacks of traditional statistical downscaling methods in regions like the Great Lakes where lake-atmosphere processes driving energy, momentum, and moisture fluxes are critical and likely nonstationary under climate change (Lanzante and others, 2020; Briley and others, 2021). However, large changes in modeled precipitation and temperature because of lake model coupling are often detected overlake and to the north and east of the Great Lakes, which is outside the domain for this study (Bennington and others, 2014; Mallard and others, 2014; Xiao and others, 2016). Where appropriate, projections of change are compared with dynamical downscaling literature to provide a more complete picture of regional change. This comparison includes analysis of two recent dynamical downscaling efforts: an ensemble downscaled using RegCM4 (Notaro and others, 2015a, b) and the multimodel ensemble NA-CORDEX (Mearns and others, 2017).
Climate data are presented for four mean periods—a historical period (1971–2000) and three future periods (2010–39, 2040–69, and 2070–99). These 30-year periods provide a long enough climate window to complete a robust analysis of period means and changes. At each future period, projections are provided for two Intergovernmental Panel on Climate Change emissions scenarios: RCP4.5 and RCP8.5. Both of these scenarios are considered equally plausible. Future projections are presented by selecting a spread of three models that represent the 25th percentile, median, and 75th percentile change in a given variable averaged over the U.S. extent of the Great Lakes Basin (fig. 1). The minimum and maximum change models are shown in appendix 1 (figs. 1.1–1.8). These chosen models indicate a spatially consistent projection from an individual model rather than presenting a combined median and range calculated at the individual grid cells. This presentation highlights how the magnitude and the spatial pattern of changes vary among model projections. Thus, for example, the driest or warmest model in the early future period (2010–39) may not show the same climate trends as the driest or warmest model in the late period (2070–99) and this presentation reflects the different rates of change among models and over the full future period. For clarity, the model used in each panel is marked by a letter in the bottom right-hand corner of each panel, and the corresponding model is detailed in table 1.
Species Background
Lake sturgeon are long lived (50–150 years) and late maturing (mean age at maturity of 15–25 years) and may spawn intermittently, making them sensitive to disturbances like climate and land-use change (Stults and others, 2016). Additionally, lake sturgeon have multiple thermal and hydrological requirements that may limit their ability to respond to change. Lake sturgeon are potamodromous (freshwater migratory fish) and fluvial dependent, requiring drainage basins that have diverse and unobstructed habitat (>250–300 km) to support their life cycle (Bruch and others, 2016). As opportunistic feeders, lake sturgeon mostly feed on benthic prey (for example, invertebrates and fish eggs) but will also feed on pelagic prey when available (for example, zooplankton and fish; Bruch and others, 2016).
Historically, lake sturgeon experienced mass declines in the late 1800s to early 1900s after European colonization because of habitat fragmentation and extensive overharvest (Bruch and others, 2016; Stults and others, 2016). Despite the closure of commercial and recreational fisheries in many areas, lake sturgeon failed to rebound because of two primary factors: habitat fragmentation and their life history. The construction of dams has fragmented habitat to prevent access to spawning and rearing areas, reducing natural reproduction (Baker, 2006). Additionally, the lake sturgeon life history means that recruitment can be low even with large populations and may not compensate for high adult mortality (Baker, 2006; Stults and others, 2016). The history of decline, persistent effects of habitat fragmentation, and life history make lake sturgeon especially sensitive to additional human disturbance from climate change.
Spawning
Lake sturgeon may spawn intermittently (once every 2–6 years), which presents tradeoffs for population stability and resilience (Baker, 2006). Although unfavorable conditions may be avoided, intermittent spawning may result in fewer opportunities for population recovery from disturbances, leading to overall population decline. The climate connections discussed in this report focus on multidecade trends; however, interannual variability is likely and may affect spawning success in any given year.
Movement to Spawning Sites
Adult lake sturgeon migrate upstream to spawning grounds in response to environmental cues related to the interaction among river flow, water level, and water temperature (Bruch and others, 2016; Ecclestone and others, 2020). Specifically, lake sturgeon move from lakes or deeper, slower river areas to rapid river environments when river flows increase and water temperatures reach 9–18 degrees Celsius (°C; Bruch and others, 2016; Baril and others, 2018; Ecclestone and others, 2020). The rate of water temperature increase also affects spawning timing (Bruch and others, 2016). Historically, the spawning period has been between April and June for lake sturgeon in the Great Lakes region (Baril and others, 2018). Changes in precipitation amount and timing, including extreme events, can alter runoff, lake levels, stream depth, stream velocity, and the timing of peak and low flow. These changes in precipitation can affect the timing and length of the spawning season (Baril and others, 2018).
Warmer air temperature could alter lake sturgeon metapopulation dynamics, for example, resulting in changes in the spawning timing of populations in the same areas (Buchinger and others, 2022). Based on a negative correlation between temperature when spawning happens and site latitude, lake sturgeon populations may be adapted to their local climate, but further research is needed to understand these relations (Baril and others, 2018).
Streamflow
Lake sturgeon are dependent on instream conditions throughout their life cycles; therefore, variability in streamflow may affect population resilience. In the Great Lakes Basin, streamflow is projected to change in velocity and timing. Stream depth and velocity are affected by runoff, which is in turn driven substantially by precipitation amount in this region. Timing and variability of streamflow are affected if the precipitation seasonality and amount change or if snowmelt contribution is reduced or shifted earlier.
Precipitation is expected to increase annually in the basin with possible drying in the summer (June–August; Easterling and others, 2017; fig. 3A–F; appendix 1, figs. 1.1–1.4). Although many climate models and the mean of the model ensemble indicate increasing precipitation in most seasons, several plausible models project future drying, especially at the mid- and late century in theRCP8.5 scenario. In general, uncertainty in precipitation projections increases with time and emissions scenario. The U.S. Great Lakes Basin mean precipitation changes in MACA2-METDATA are listed in table 2.
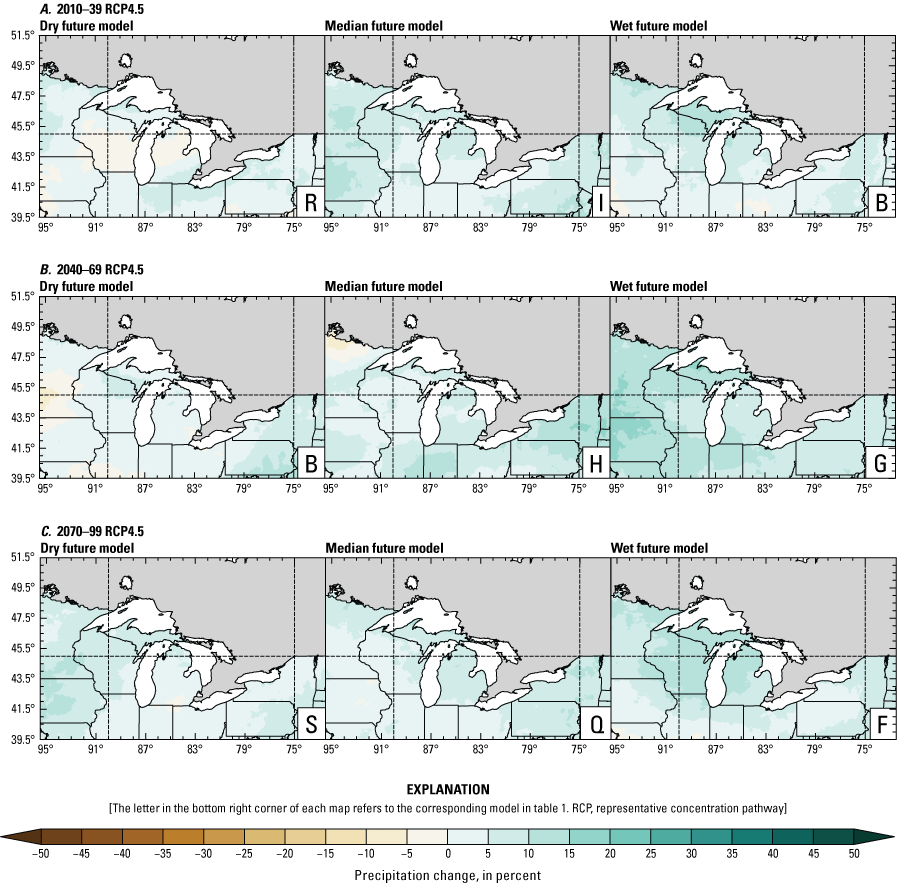
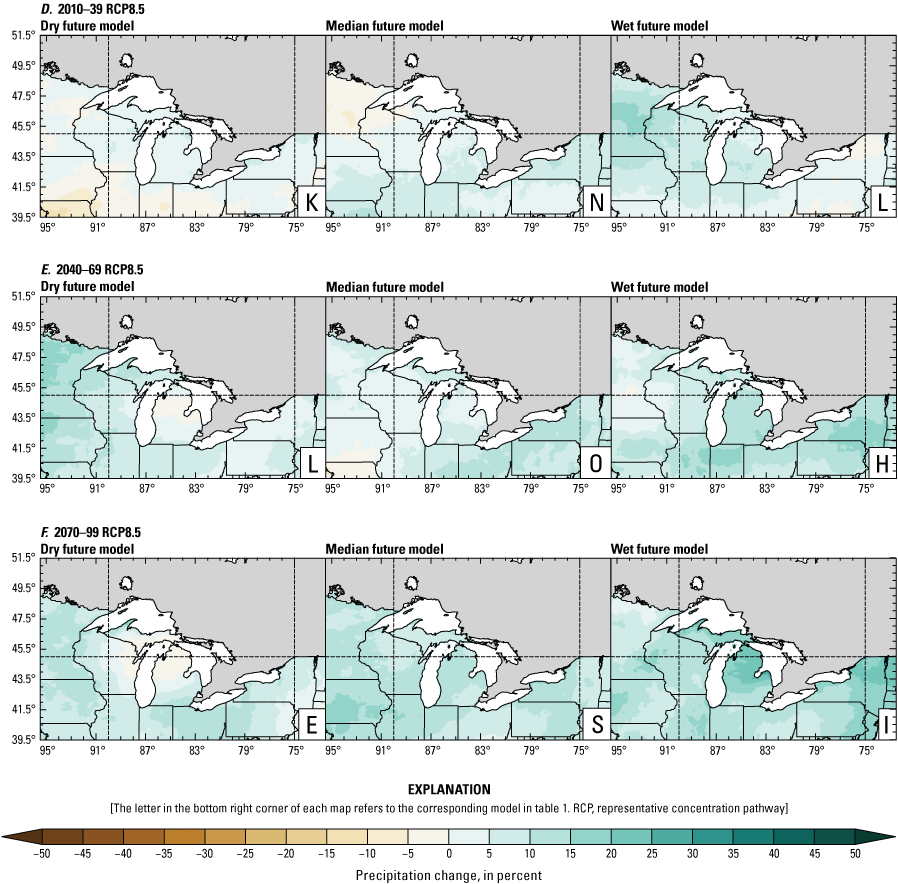
Annual mean total precipitation change (in percent) in the downscaled climate simulations under emissions scenarios for representative concentration pathway 4.5 (RCP4.5) and representative concentration pathway 8.5 (RCP8.5) from the historical period (1971–2000) to the future periods. A, early 21st century (2010–39) for RCP4.5; B, mid-21st century (2040–69) for RCP4.5; C, late 21st century (2070–99) for RCP4.5; D, early 21st century (2010–39) for RCP8.5; E, mid-21st century (2040–69) for RCP8.5; F, late 21st century (2070–99) for RCP8.5.
Table 2.
Change in seasonal and annual precipitation (in percent) averaged over the U.S. Great Lakes Basin from the historical period (1971–2000) for the three future periods under representative concentration pathways 4.5 and 8.5 using Multivariate Adaptive Constructed Analogs version 2 downscaled global climate model data (Abatzoglou and Brown, 2012). Median model values are given.[Bold values indicate range spans of zero. The range between the wettest and driest model is given in parentheses. RCP, representative concentration pathway]
The projections documented in table 2 are largely consistent with recent literature projecting increases in annual precipitation, more consistent increases in spring (March–May), and a possibility of decreased precipitation in the summer (Notaro and others, 2015b; Mailhot and others, 2019; Channell and others, 2022a, b, c, d; Kayastha and others, 2022; Shrestha and others, 2022; Xue and others, 2022). Variations in projections can be attributed to differences in area considered, periods, and model variability. The high likelihood of increased precipitation in the winter and spring is important because it may contribute to earlier and increased streamflows.
In contrast, the depth of snowpack is projected to decrease with earlier snowmelt because of increasing air temperatures (Dyer and Mote, 2006; Notaro and others, 2011; Mailhot and others, 2019; Shrestha and others, 2022). This melt timing may push snowmelt-associated peak streamflow earlier (Dudley and others, 2017; Byun and others, 2019; Dhakal and Palmer, 2020) while reducing the number of snowmelt-driven peak events and the amount of snowmelt runoff (Notaro and others, 2013; Burn and Whitfield, 2023). Additionally, flooding events may decrease because of reduced snowpack but, when they do happen, are likely to happen earlier in the year (Li and others, 2019). This finding is consistent with historical data for some areas west of Lake Michigan (not shown) where annual streamflow has decreased between 1960 and 2015 (Norton and others, 2019), although another study indicates no consistent trends from 1951 to 2009 (McCabe and Wolock, 2014).
Overall, changing precipitation seasonality, increasing precipitation in most seasons (with more precipitation falling as rain versus snow in most areas), reduced snowpack amount, and altered snowmelt timing may concentrate high flow events earlier in the season. Decreases or increases in spring flow are dependent on snowmelt and rainfall, but flow may increase with increases in winter and spring precipitation. In general, projections point to increases in winter and spring runoff in the Great Lakes Basin, coincident with increased precipitation and earlier snowmelt (Notaro and others, 2015a; Mailhot and others, 2019; Channell and others, 2022a, b, c, d; Kayastha and others, 2022). Lake sturgeon spawning may be affected by increased spring flows because this season is a critical reproductive period. Earlier and more intensive high flow events may result in shifted spawning timing for adult lake sturgeon leading to unusual or protracted spawning events, inaccessible habitat, and unsuitable conditions for eggs and larvae (see more in the “Spawning and Egg Survival” section).
Water Temperature
Increased lake and stream temperatures, especially in the spring, resulting from changes in air temperature, ice cover, shifting stratification, and snowmelt may lead to earlier spawning and prolong the exposure of lake sturgeon to warmer conditions. Climate change may lead to increased air temperatures across all seasons for the full range of lake sturgeon habitats (fig. 4A–F; appendix 1, figs. 1.5–1.8). Northern basins (for example, Ontario) are expected to warm more than southern areas in the winter and spring, whereas southern and central areas (for example, Michigan) are expected to warm more in the summer. Air temperatures in the northern Great Lakes Basin have already increased more than other parts of the basin, with the largest increases in the winter (Vose and others, 2017). Models consistently project these changes, with winter continuing to warm more than other seasons (fig. 4A–F; appendix 1, figs. 1.5–1.8; Xue and others, 2022). The Great Lakes Basin mean temperature change in MACA2-METDATA is listed in table 3.
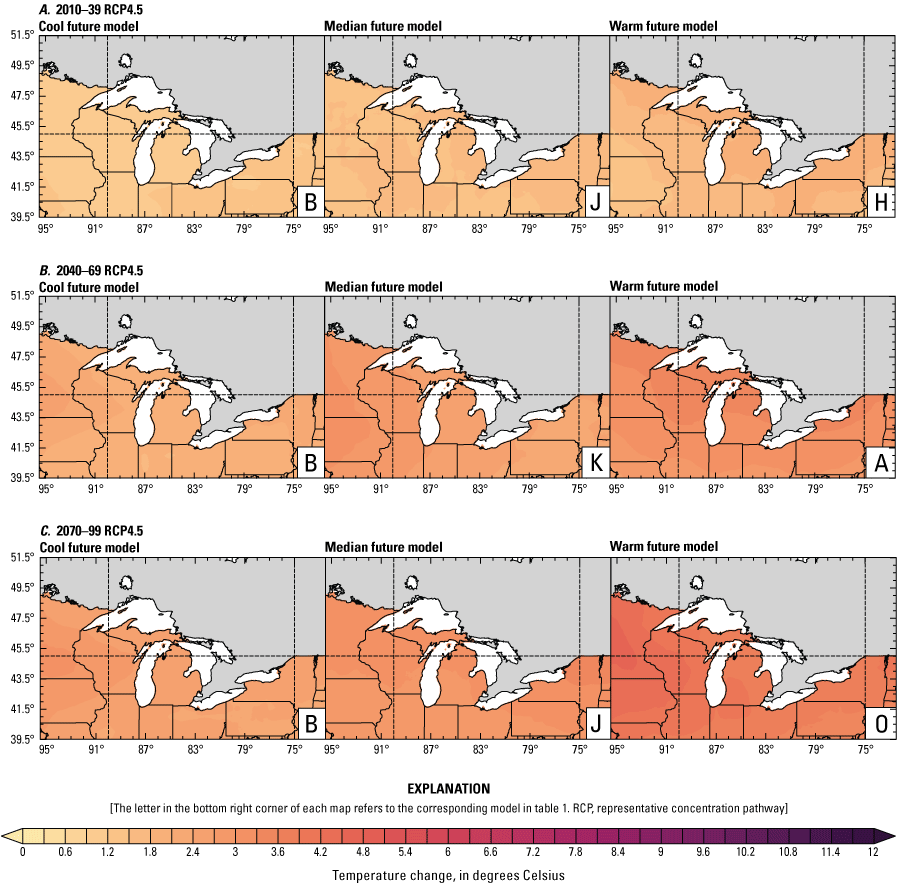
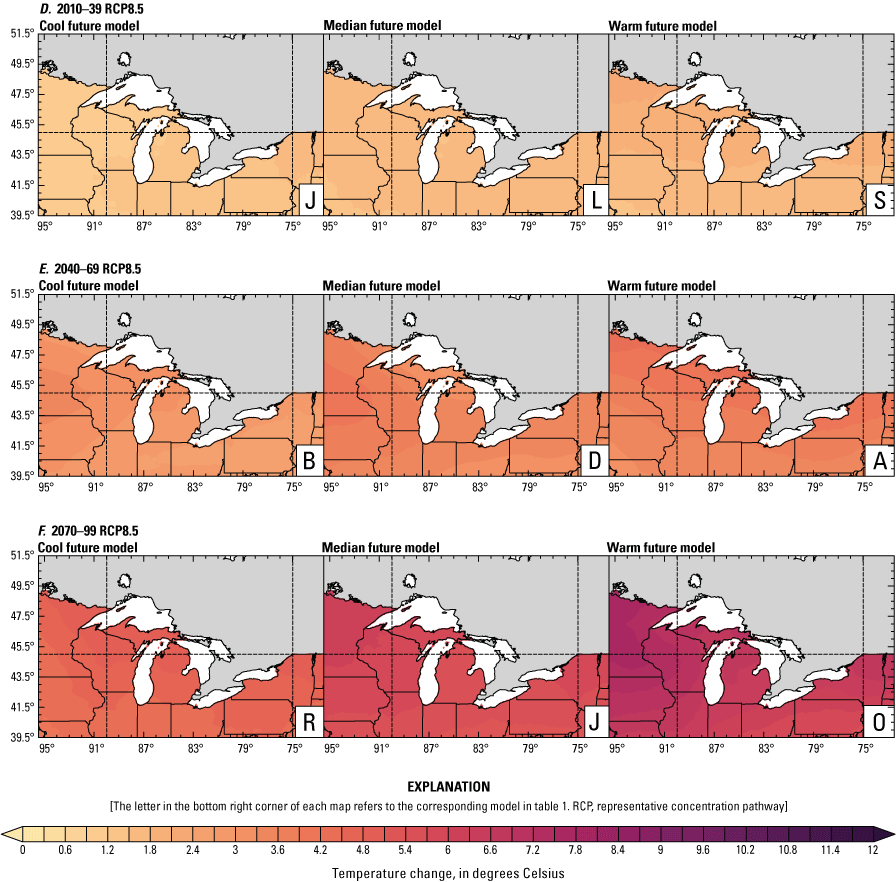
Annual mean 2-meter air temperature change (in degrees Celsius) in the downscaled climate simulations under emissions scenarios for representative concentration pathway 4.5 (RCP4.5) and representative concentration pathway 8.5 (RCP8.5) from the historical period (1971–2000) to the future periods. A, early 21st century (2010–39) for RCP4.5; B, mid-21st century (2040–69) for RCP4.5; C, late 21st century (2070–99) for RCP4.5; D, early 21st century (2010–39) for RCP8.5; E, mid-21st century (2040–69) for RCP8.5; F, late 21st century (2070–99) for RCP8.5.
Table 3.
Change in seasonal and annual temperature (in degrees Celsius) averaged over the Great Lakes Basin from the historical period (1971–2000) for the three future periods under representative concentration pathways 4.5 and 8.5 using Multivariate Adaptive Constructed Analogs version 2 downscaled global climate model data (Abatzoglou and Brown, 2012). Median model values are given.[The range between the coolest and warmest model is given in parentheses. RCP, representative concentration pathway]
Earlier ice melt is linked to warming air temperatures. Historically, total ice cover has decreased. Although this change can be quantified linearly as a decrease of 71 percent from 1973 to 2010 in the Great Lakes region (Wang and others, 2012), it is more appropriate to view the change as a regime shift from high mean ice cover (69 percent) to low mean ice cover (36 percent) in 1998 (Van Cleave and others, 2014). Ice-out dates happened 2–5 days earlier over the 1950–2012 period, and the freeze-free season has lengthened on inland lakes across northeastern Minnesota (Stults and others, 2016); however, low ice in one year can lead to high ice conditions the next year by modifying warming and evaporation rates (Wang and others, 2012; Spence and others, 2013). Additionally, natural variability and very cold winters can contribute to higher ice cover in some years (Wang and others, 2012; Spence and others, 2013); therefore, projections of decreased mean ice coverage and duration will be accompanied by strong interannual variability (Austin and Colman, 2007; Xue and others, 2022).
Earlier ice-free days and resulting earlier stratification are likely to lead to increased spring lake temperatures (Xue and others, 2022). Although lake temperatures may not be substantially affected by spring runoff, reduced contribution of snowmelt to streamflow may increase the diurnal variability and mean of stream temperature and increase sensitivity to changing air temperatures (Constantz, 1998; Ficklin and others, 2013; Lisi and others, 2015). Warming spring water temperatures are a key spawning signal for adult lake sturgeon to begin migratory movements; therefore, these changes may combine with other signals (for example, high flows) to trigger earlier and potentially prolonged spawning periods.
Spawning and Egg Survival
Lake sturgeon spawning typically happens on unobstructed gravel shoals and stream rapids from April to June (Stults and others, 2016). From a lake sturgeon range-wide assessment, four habitat characteristics affect spawning success: flow velocity, water depth, water temperature, and substrate size (Baril and others, 2018). Specifically, peak spawning suitability was observed at velocities of 0.6 meter per second, depths of 0.55–0.85 meter in small rivers (less than [<] 100 cubic-meter-per-second mean annual streamflow) and 0.75–5.25 meter in large rivers (>100 cubic meters per second), and over cobble, and suitable water temperatures decreased with increasing latitude (Baril and others, 2018). Once released, eggs drift downstream and yolk-sac larvae emerge about 11–19 days after postpeak spawn, sometimes drifting more than 61 km downstream (Baker, 2006; Bruch and others, 2016). Eggs may have limited hatch success when impeded by sediment or algae (Bruch and others, 2016).
Extreme Events and the Interaction Between Flow and Temperature
Flow and runoff in the Great Lakes Basin are projected to increase in the spring and potentially decrease in the summer, with the potential for increased extreme flood and drought events. Historically, precipitation associated with heavy rainfall events has increased, and more of the increase is associated with higher frequency of events than intensity (Mallakpour and Villarini, 2015; Hoerling and others, 2016). In the Midwest, extreme precipitation events have increased over time, especially in the autumn, with a 42-percent increase in extreme precipitation (99th percentile) from 1958 to 2016 (Easterling and others, 2017). Continued warmer temperatures are expected to contribute to increased heavy precipitation events in the future, although interactions between increasing and variable temperatures and precipitation are difficult to quantify, especially considering model overestimation of historical winter precipitation (Diffenbaugh and others, 2013; Feng and others, 2016; Notaro and others, 2021).
Extreme precipitation and temperature events have a substantial effect on instream conditions experienced by lake sturgeon and their prey. Extreme precipitation events can cause an influx of contaminants and sediments, especially for sites near developed areas, which may coincide with lake sturgeon spawning locations. Large precipitation and runoff events may flush fertilizer and other effluent from surrounding agricultural areas into stream basins. Toxic effluents have been cited as a source of lake sturgeon mortality in some sites such as the St. Lawrence River (Dumont and others, 1987). Effluents also indirectly affect juvenile and adult lake sturgeon by decreasing forage availability (see more in the “Larvae and Juvenile Survival” section; Dumont and others, 1987).
Lake sturgeon eggs may be buried with increased sedimentation from extreme events, resulting in reduced hatching success (Bruch and others, 2016; Stults and others, 2016). In addition, lake sturgeon are vulnerable to low oxygen conditions that may result from reduced water quality (Harkness and Dymond, 1962). Extreme high and low flow events can contribute to flushing or desiccation risk of eggs. In general, decreased streamflow is associated with decreased survival, growth, and abundance of fish populations and shifts in community composition; therefore, the increased variability in streamflows associated with climate change may result in adverse conditions for lake sturgeon (van der Lee and others, 2022).
Although runoff and streamflow may increase in spring because of increasing precipitation and shifting snowmelt, summer flows may be reduced because of a possible reduction in precipitation, increasing temperatures, and earlier snow melt. Increased air temperatures and heat waves can cause rapid increases in stream temperature. Daily maximum temperatures have increased in the Great Lakes Basin and are projected to become more frequent, with a substantial increase in the number of extremely hot days (Lopez and others, 2018; Zhang and others, 2018; Xie and others, 2021). In addition to signaling the start of spawning migrations, flow variability and water temperature also provide cues for spawning cessation (Baril and others, 2018). Lake sturgeon tend to spawn as river flow decreases and will cease spawning activity if streamflow or water temperature change rapidly (Baril and others, 2018); therefore, increased variability in river flows and temperature conditions may result in abbreviated spawning events with uncertain outcomes for egg and larval survival.
For lake sturgeon, outmigration to a lentic environment could be a response to potentially uncertain conditions, ensuring that fish are not stranded in unfavorable conditions such as complete dewatering or extensive freezing (Ecclestone and others, 2020). If climatic changes lead to lower river levels, spawning areas could become inaccessible to fish, along with reduced overall suitable habitat (Stults and others, 2016). For example, flash droughts caused by high temperatures and low precipitation (Mo and Lettenmaier, 2020) could contribute to spawning period stranding, spawning cessation caused by a rapid change in conditions, or a potential transition to other spawning locations (Moore and others, 2022).
General Water Conditions
Maintenance of adequate river conditions during spawning season is crucial for lake sturgeon reproductive success. Results demonstrate that the mean and variability in temperatures experienced during egg incubation have considerable effects on larval body size and timing of development whereas increasing mean stream temperatures have mixed effects on spawning success (Dammerman and others, 2019). Mean June air temperature was positively correlated with increased abundances of young lake sturgeon (Adams and others, 2006). This correlation may be linked to food availability because early lake sturgeon life stages are dependent on zooplankton and benthic invertebrates; however, increased temperatures are linked to increased fungal transmission to eggs and may promote the development of benthic algal blooms, resulting in reduced hatching success (Bruch and others, 2016). Therefore, projected increases in temperature may result in enhanced growth and survival of larval lake sturgeon under certain conditions but may adversely affect egg hatching and larval development in other contexts when optimal thresholds are exceeded.
Spawning Conclusions
Given the importance of riverine habitats for lake sturgeon throughout various life stages, shifting streamflow may have implications for adult spawning timing and success. Increasing winter and spring precipitation in combination with earlier snowmelt may result in earlier spring high streamflow events. Spring high streamflow events are key spawning signals for adult lake sturgeon to make migrations from deep-water habitats to fast-flowing, shallow spawning areas; therefore, earlier high streamflow events may result in earlier and prolonged flows suitable for spawning for lake sturgeon. Additionally, increased water temperature resulting from increased air temperatures, less ice and earlier melt times, and earlier spring stratification could also trigger earlier spawning. Although earlier spawning events may result in increased growth periods for eggs and larvae, conditions may not be favorable to support successful egg and larval development given adverse effects from increased extreme events (for example, flood waters scouring eggs). Further, an earlier spawning season, in combination with increased variability in spring conditions (for example, increased extreme precipitation events), could lead to more frequent cues to cease spawning, resulting in condensed spawning events.
High or extreme flow conditions can also lead to increased sedimentation and contaminant influxes, which could adversely affect lake sturgeon spawning ability and be detrimental to prey. Reduced summer precipitation and increased temperatures may lead to low flow conditions and hot periods in some years, which can cue spawning cessation, negatively affecting lake sturgeon and prey, and contribute to algal blooms, leading to reduced egg hatch success. Increased variability in extreme events has the potential to limit habitat availability for adult lake sturgeon and present challenges for egg survival; however, in systems where ample habitat is available, spawning site fidelity has been documented to be weak, and fish may spawn in numerous locations and over protracted periods (Bruch and others, 2016). Diversified spawning sites may lead to increased resilience to protect against adverse conditions in a single year or location (Forsythe and others, 2011; Paradis and others, 2022). The effect shifted spawning timing and conditions may have on population growth, individual body condition, and survival is unclear and highlights key knowledge gaps.
Larvae and Juvenile Survival
After hatching and moving downstream, young lake sturgeon primarily inhabit shallow nearshore wetland and marsh habitat. Water quality issues (for example, nutrients from agricultural runoff, contaminants from industry, and algal blooms) are a particular concern for nearshore areas, especially under low lake-level conditions and changes in the amount and timing of runoff (Abdel-Fattah and Krantzberg, 2014). Given potential increased variability in water levels, wetland water quantity and quality may be adversely affected such that suitable lake sturgeon nursery habitat is reduced.
Larval Movement
Larvae hatch from eggs soon (11–19 days) after egg release and are dependent on flows for movement downstream to nursery sites. Therefore, larval lake sturgeon are dependent on similar water temperature and flow dynamics as spawning conditions. As a result of more variable and earlier peak flows, larvae may be subjected to additional challenges finding suitable nursery habitat because of extreme precipitation events.
Habitat Requirements
Rapid change and interannual variability in Great Lakes Basin lake water levels can cause ecosystem disturbance to crucial larval and juvenile nursery habitat. Lake levels are dictated by the components of net basin supply (NBS; the balance among overlake precipitation, evaporation, and runoff; Deacu and others, 2012). Rising lake levels in the spring from increasing runoff from precipitation and snowmelt are sustained during the summer by low evaporation and decrease in the autumn as evaporation reaches its annual peak.
Great Lakes water levels have gone through several periods of decrease and increase in the last 50 years, with substantial reductions until 2014 when water levels began to rise (Assel and others, 2004; Gronewold and others, 2016; Gronewold and Rood, 2019). The latest models, which have the benefit of dynamical downscaling (Notaro and others, 2015b), lake model coupling (Xue and others, 2017, 2022; Sharma and others, 2018; Notaro and others, 2021; Kayastha and others, 2022), and more accurate energy balance considerations for lake evaporation (Lofgren and others, 2011; Lofgren and Rouhana, 2016), project stable or increasing mean lake levels in the future (Mailhot and others, 2019; Channell and others, 2022a, b, c, d; Kayastha and others, 2022; VanDeWeghe and others, 2022). Runoff seasonality shifts may also lead to an earlier annual increase in lake levels (see more in the “Streamflow” section; VanDeWeghe and others, 2022).
Although the mean change in lake levels is less certain, the interannual variability of the NBS, and consequent lake levels, is likely to increase in the future (Gronewold and Rood, 2019; Seglenieks and Temgoua, 2022; VanDeWeghe and others, 2022). Extreme low and high water levels may have positive effects on coastal habitat by promoting wetland plant germination and preventing desiccation, respectively (Assel and others, 2004). Extended periods of low water levels or rapid changes in water level, however, may cause degradation of nearshore habitat. Ultimately, evidence indicates that the intensity and rate of fluctuations in lake levels can be harmful to nearshore habitats, and planning for potential increased variability may be critical (Gronewold and Rood, 2019; Theuerkauf and Braun, 2021).
Nearshore ice cover is important to insulate and decrease evaporation in nearshore areas. Sublake-level analysis indicates that ice coverage has decreased more quickly in nearshore areas (Mason and others, 2016) and is generally projected to decrease with strong variations from year to year (Xiao and others, 2016; Xue and others, 2022). Ice cover duration has important implications for lake temperature, the rate of warming, and resulting stratification. Increased air temperatures and decreasing lake ice are likely to lead to earlier and more rapid warming in the spring and summer, which could reduce suitable habitat for juvenile lake sturgeon and lead to increased mortality (see more in the “Water Temperature” section).
Indirectly, shifting water temperatures can affect food availability and cause a zooplankton phenological mismatch when larval lake sturgeon hatch before zooplankton emergence and do not have access to this important food source. Increased temperatures (sometimes from reduced ice cover and water levels) in nearshore areas may also increase diel hypoxic events, resulting in adverse habitat conditions for larvae and juveniles (Tellier and others, 2022).
Larvae and Juvenile Survival Conclusions
The direct and indirect effects of changing ice cover, water levels, and lake temperatures are likely to affect larval and juvenile lake sturgeon in the future. Climate change is likely to affect lake sturgeon larvae and juvenile survival through changes in development timing, habitat conditions, and prey availability. Increasing water temperatures and earlier spring precipitation events may cause earlier hatching, with uncertain consequences for lake sturgeon development and growth. Further, shifts in hatch timing may be misaligned with prey emergences (for example, zooplankton), resulting in decreased food availability for early life stages.
Nearshore wetlands provide key nursery habitats for larval and juvenile lake sturgeon, but increased spring extreme events may flush eggs and larvae away from these habitats. Indirectly, changes in precipitation and decreased ice cover may lead to lower and more variable water levels, further reducing the availability of suitable refuge habitat for larvae and juveniles. The effect projected changes in precipitation, water levels, temperature, and winter conditions may have on early life stages of lake sturgeon is unclear; therefore, considering the interactions among these factors when assessing future habitat conditions and potential refuge is beneficial.
Adult Foraging and Survival
After spawning, adults commonly migrate back to deeper slow flow areas in lakes and rivers (Ecclestone and others, 2020) where they spend most of their life. Higher water temperatures may cause acute mortality or increase stress and thereby affect condition, growth, and mortality rates for adult lake sturgeon. Warmer water temperatures may also negatively affect benthic productivity, specifically of cool-water invertebrates, and increase the incidence of parasites and diseases of sturgeon (Haxton, 2008); however, some prey items, including warm-water invertebrates, may benefit from warmer water temperatures, resulting in increased food resources for lake sturgeon.
Physiological Preference and Limits
Lake sturgeon are a cool-water fish, where adults prefer water temperatures <25 °C (Cech and Doroshov, 2004) and have an optimal temperature for growth at <20 °C (Haxton and others, 2016). Lake sturgeon are highly mobile (for example, spawning migrations >100 km) and spend limited time in hypolimnetic waters (deep areas below the thermocline); therefore, lake sturgeon are more likely to be exposed to warming surface temperatures.
Lake temperatures in the Great Lakes Basin are expected to warm in the future, although warming will not be equal across lakes. Increases in lake surface temperature (LST) are related, but not equal, to increases in air temperature. For example, LST in Lake Superior increased at double the rate of the overlying air temperature from 1979 to 2006 (Austin and Colman, 2007; O’Reilly and others, 2015). Projections agree that the Great Lakes will continue to warm in the future, but the rate of that warming remains uncertain (Zhong and others, 2016) and requires a broader set of investigations using coupled lake modeling. Warming temperatures, a potential reduction in lake ice, and an earlier onset of thermal lake stratification are expected to enhance summer LST warming (Austin and Colman, 2007; Zhong and others, 2016). Model projections indicate that annual mean lake temperature increases will continue to be higher than surrounding air temperature increases (table 4; Xue and others, 2022). Despite recent warming, LST temperatures are not projected to exceed optimal and preferred ranges for lake sturgeon on average in the summer except slightly in Lake Erie; however, extreme heat events in any given year can further boost summer LST greater than the ranges preferred by lake sturgeon (table 4). Linear trends in LST are complicated by short data spans and strong spatial heterogeneity in warming within the lakes (Mason and others, 2016).
Table 4.
Historical and projected summer mean lake surface temperatures.[LST, lake surface temperature; GLERL/GLSEA, Great Lakes Environmental Research Laboratory/Great Lakes Surface Environmental Analysis; RCP, representative concentration pathway]
Region | 1995–2021 mean summer LST (GLERL/GLSEA)1, in degrees Celsius | Historical summer lake warming trends, in degrees Celsius per year; period; and reference | End of century (2000–19 to 2080–99) change in LST2, in degrees Celsius, and RCP model |
---|---|---|---|
Lake Superior | 11.4 | 0.09, (1979–2006), Austin and Colman (2007) 0.14, (1994–2013), Mason and others (2016) |
3.2 (RCP4.5) 6.1 (RCP8.5) |
Lake Ontario | 19.1 | 0.05, (1968–2002), Dobiesz and Lester (2009) 0.1, (1994–2013), Mason and others (2016) |
2.5 (RCP4.5) 5 (RCP8.5) |
Lake Huron | 16.8 | 0.08, (1968–2002), Dobiesz and Lester (2009) 0.09, (1994–2013), Mason and others (2016) |
2.5 (RCP4.5) 4.5 (RCP8.5) |
Lake Erie | 21.7 | 0.06, (1994–2013), Mason and others (2016) | 2 (RCP4.5) 4 (RCP8.5) |
Lake Michigan | 17.9 | 0.09, (1994–2013), Mason and others (2016) | 2.5 (RCP4.5) 4.5 (RCP8.5) |
Historical LST available for download at https://coastwatch.glerl.noaa.gov/statistic/.
End of century change in LST data are from Xue and others (2022).
Increased water temperature affects ice cover, evaporation, and stratification onset and duration. Historically, more northern and deeper lakes increasingly warmed in part because of shifting stratification dynamics and shallow lakes warmed from increased spring temperatures and solar radiation (Zhong and others, 2016). Lake stratification affects water circulation and hypolimnetic oxygen availability and amplifies warming. Additionally, high water temperatures can combine with low oxygen levels to cause mass fish mortality events, reducing population abundances and resilience (Hupfeld and others, 2015). Although the Great Lakes Basin is projected to warm, these dynamics will likely not be uniform amongst and within a given lake given interactions between ice cover and stratification, which may affect conditions for organisms such as lake sturgeon.
Effect on Activity
Lake sturgeon are considered a cool-water fish but can live in water temperatures warmer than most of their current habitat (Moore and others, 2022). Warmer temperatures may increase the abundance of lake sturgeon in some areas in which they were once limited (for example, northern Canada; Lemmen and Warren, 2004). Therefore, apart from the shallowest inland lakes and rivers, the projected near-term warming may extend the growing season and increase productivity for lake sturgeon, although indirect effects in timing of reproduction and overall ecosystem health could result in adverse effects (Magnuson and others, 1997; Stults and others, 2016). Most river-lake networks have cooler segments, large tributaries, or lakes that might provide temporary escape from potentially less suitable temperatures (Lyons and Stewart, 2014). Temperature also affects individual movement, with populations at lower latitudes and those that experience warmer temperatures having lower summer movement relative to spring movement, potentially indicating local adaptation or behavioral plasticity (Moore and others, 2021). Understanding suitable thermal habitat given climate change projections can inform how lake sturgeon may respond to further warming.
Food Availability
As opportunistic, benthic feeders, juvenile and adult lake sturgeon mostly feed on invertebrates and fish eggs, although they may feed on pelagic prey when available (for example, zooplankton and fish; Bruch and others, 2016). Increasing temperatures and associated changes to oxygen may affect the phenological timing and abundance of important prey (for example, zooplankton; Magnuson and others, 1997; Adams and others, 2006); therefore, the seasonal dynamics of projected climate changes in relation to lake sturgeon life stages are important to consider.
Changes to water temperature and precipitation may increase algal blooms, which may exacerbate low oxygen availability caused by an extended stratification period in deep ponds and even in shallower waterbodies that do not achieve full seasonal stratification (Chapra and others, 2017; Shinohara and others, 2023). Algal blooms may also be affected by changes in flow regime and associated inputs of nutrients and contaminants (Chapra and others, 2017). Algal blooms and changing stratification dynamics may lead to food web shifts such that lake sturgeon are indirectly affected by limnological changes in areas they do not directly inhabit.
Other Biotic Interactions
Rising temperatures can also contribute to higher parasite and disease transmission (Haxton, 2008) and increased interaction with nonnative species. Effects of nonnative species on lake sturgeon are somewhat unknown and difficult to predict, but species introductions could affect lake sturgeon populations through shifts in the food web or alterations to habitat. For lake sturgeon, Dreissena polymorpha (Pallas, 1771; zebra mussels) and Neogobius melanostomus (Pallas, 1814; round goby) are of particular concern given their increasing prevalence in some parts of the Great Lakes Basin (Escobar and others, 2018). Climate change effects on river flow (Rahel and Olden, 2008) and temperature may make habitats more suitable for nonnative species, increasing their abundances and distributions leading to further interactions with native fishes such as lake sturgeon (Escobar and others, 2018). Nonnative species may increase competition for prey and directly predate on different lake sturgeon life stages. For example, round gobies have preyed on lake sturgeon eggs, reducing hatch success (Kornis and others, 2012).
Newly introduced species may provide an alternate food source. Zebra mussels may affect the feeding efficiency of lake sturgeon (McCabe and others, 2006) but can also contribute an important dietary component for lake sturgeon larger than >70 centimeters (Jackson and others, 2002). Further, round gobies can be a preferred bait species for lake sturgeon indicating round goby may be a preferred food source (Thomas and Haas, 2002); therefore, lake sturgeon increases in round goby may inadvertently benefit lake sturgeon. Lake sturgeon, being benthivores (feeding predominantly on bottom resources), may offset the negative ecological effects of some nonnative introductions if lake sturgeon populations were of substantial size (Haxton, 2008), but these novel interactions are a key knowledge gap.
Adult Foraging and Survival Conclusions
Climate change has the potential to affect adult foraging and survival in positive and negative ways. Changes in water basin levels and thermocline depth, ice cover reductions, LST increases, summer stratification duration, and hypolimnetic hypoxia increases are projected for the future. These changes may directly affect fish, prey, habitat, and other biotic interactions (Mulvaney and others, 2014). Effects of climate change (for example, warming water temperatures) may lead to increased individual growth and nonnative prey availability for adult lake sturgeon; however, this growth may be offset by losses in native prey and limited habitat availability. Specifically, further research is needed on the potential for algal blooms and hypoxia in deeper lakes; much of the current research is focused on shallower lakes, particularly Lake Erie (Tellier and others, 2022). Given the potential effects of climate change, whether lake sturgeon will have viable habitat in the future and whether that habitat will align with historical occupancy are two questions with varying implications.
Conclusions
Adult lake sturgeon span multiple habitats during their long lifespans, including high gradient streams, nearshore areas, and deep rivers and lakes (Moore and others, 2021). However, climate change is projected to strongly affect the suitability of these habitats through increasing precipitation and temperatures and decreasing ice cover and snowmelt. These changes may lead to myriad effects on lake sturgeon, including changes in spawning initiation and cessation, egg survival, larval growth, juvenile foraging, and adult movement; however, the effects of the interactions of complex climate drivers on lake sturgeon populations across their range is still unclear.
Higher air temperatures are projected to coincide with reduced ice cover to drive increases in LST, stream temperature, and lake stratification, which may contribute to increased hypoxia and algal blooms. These changes are linked to altered mortality risk and prey availability. Changes in precipitation and snow seasonality, volume, and distribution of extreme events affect streamflow, stream temperature, runoff, and lake levels. These changes are linked to movement and hatching success of eggs and larvae and availability of larval and juvenile nursery habitat. All projected changes are likely to affect spawning timing and duration.
Many of these changes are already being observed, especially in communities where lake sturgeon are economically and culturally important. For example, indigenous fishers and managers have noticed shifts to shorter winters and reduced ice cover on Lake Superior over their careers, contributing to warmer water temperatures, earlier ice out on inland lakes, shifting species compositions in lakes, increasing nonnative species, and an alteration in precipitation timing and intensity (Stults and others, 2016). Despite an understanding of historical and future climatic conditions for the Great Lakes Basin, the effect on lake sturgeon that may be caused by the interaction between shifting dynamics is still unclear.
Several gaps in knowledge have been highlighted in this report that would aid in linking climate drivers to potential ecological responses for lake sturgeon. First, a deeper understanding of the potential adaptive capacity of lake sturgeon across spatial and temporal scales is needed. Lower latitude populations spawn at higher temperatures, but it is unclear if higher latitude populations may adapt to spawning at higher temperatures, especially given the rapid pace of climate change.
Other gaps in knowledge include the following:
-
How climate effects on one life stage (for example, a loss of juveniles or larvae in a given spawning year) propagate to overall population trajectories.
-
The interaction between increased precipitation and variability on sedimentation and runoff in known spawning areas, which may affect adult spawning habitat and egg hatching success.
-
The potential for and effects of nonnative species as a food source and predator, which may affect juvenile and adult abundance, growth, and condition.
-
The effect of shifting spawning timing and duration on population dynamics and prey availability, which may affect larval, juvenile, and adult abundance, growth, and condition.
-
The effect of changing water levels and reduced ice cover on future wetland conditions, which may affect larval, juvenile, and adult abundance, growth, and condition.
Despite the potential for adverse effects of climate change, some projected shifts may benefit lake sturgeon. Many populations currently live in areas where water temperatures are generally less than their preferred or optimal ranges; therefore, increased temperatures may increase the activity, movement, and prey availability in some areas and promote growth. Earlier snowmelt and peak flow events may lengthen the spawning season in some years. Additionally, lake levels are not likely to drop substantially, sparing wetland habitats from this stressor. Identifying locations where adverse climate effects may be limited could provide opportunities for increased population resilience.
Additional local drivers of change can interact with climate effects to exacerbate shifting dynamics; climate shifts may allow agricultural expansion to formerly unsuitable areas for certain crops and this and other land-use changes may interact with climate drivers (temperature and precipitation) to affect runoff of contaminants and sediment into waterbodies (Chu and Jones, 2011; Burn and Whitfield, 2023). Flow modification using dams, a potential reaction to hydrologic variability or increased anthropogenic water demand, can separate lake sturgeon from spawning grounds. In many areas, lake sturgeon have strong spawning site fidelity and return to their natal river, but dams have fragmented habitat to prevent access to spawning and rearing areas, reducing natural reproduction (Baker, 2006; Bruch and others, 2016). Further, water releases from control structures can affect streamflow, oxygen, and water temperature, which may have subsequent effects on spawning behavior and egg and larval survival (Haxton, 2008). Additional local drivers such as industrial development, deforestation, and groundwater withdrawal may affect habitat suitability for lake sturgeon (Chu and Jones, 2011). Lake sturgeon are a culturally, ecologically, and economically important fish to the Great Lakes Basin; therefore, understanding how climate may interact with other drivers is necessary to support population adaptation and resilience.
References Cited
Abatzoglou, J.T., and Brown, T.J., 2012, A comparison of statistical downscaling methods suited for wildfire applications: International Journal of Climatology, v. 32, no. 5, p. 772–780. [Also available at https://doi.org/10.1002/joc.2312.]
Abdel-Fattah, S., and Krantzberg, G., 2014, A review—Building the resilience of Great Lakes beneficial uses to climate change: Sustainability of Water Quality and Ecology, v. 3–4, p. 3–13. [Also available at https://doi.org/10.1016/j.swaqe.2014.11.006.]
Adams, W.E., Jr., Kallemeyn, L.W., and Willis, D.W., 2006, Lake sturgeon population characteristics in Rainy Lake, Minnesota and Ontario: Journal of Applied Ichthyology, v. 22, no. 2, p. 97–102. [Also available at https://doi.org/10.1111/j.1439-0426.2006.00725.x.]
Arora, V.K., Scinocca, J.F., Boer, G.J., Christian, J.R., Denman, K.L., Flato, G.M., Kharin, V.V., Lee, W.G., and Merryfield, W.J., 2011, Carbon emission limits required to satisfy future representative concentration pathways of greenhouse gases: Geophysical Research Letters, v. 38, no. 5, article L05805, 6 p., accessed April 17, 2023, at https://doi.org/10.1029/2010GL046270.
Assel, R.A., Quinn, F.H., and Sellinger, C.E., 2004, Hydroclimatic factors of the recent record drop in Laurentian Great Lakes water levels: Bulletin of the American Meteorological Society, v. 85, no. 8, p. 1143–1152. [Also available at https://doi.org/10.1175/BAMS-85-8-1143.]
Austin, J.A., and Colman, S.M., 2007, Lake Superior summer water temperatures are increasing more rapidly than regional air temperatures—A positive ice-albedo feedback: Geophysical Research Letters, v. 34, no. 6, article L06604, 5 p., accessed April 15, 2023, at https://doi.org/10.1029/2006GL029021.
Baril, A.-M., Buszkiewicz, J.T., Biron, P.M., Phelps, Q.E., and Grant, J.W.A., 2018, Lake sturgeon (Acipenser fulvescens) spawning habitat—A quantitative review: Canadian Journal of Fisheries and Aquatic Sciences, v. 75, no. 6, p. 925–933. [Also available at https://doi.org/10.1139/cjfas-2017-0100.]
Bennington, V., Notaro, M., and Holman, K.D., 2014, Improving climate sensitivity of deep lakes within a regional climate model and its impact on simulated climate: Journal of Climate, v. 27, no. 8, p. 2886–2911. [Also available at https://doi.org/10.1175/JCLI-D-13-00110.1.]
Bentsen, M., Bethke, I., Debernard, J.B., Iversen, T., Kirkevåg, A., Seland, Ø., Drange, H., Roelandt, C., Seierstad, I.A., Hoose, C., and Kristjánsson, J.E., 2013, The Norwegian Earth System Model, NorESM1–M—Part 1—Description and basic evaluation of the physical climate: Geoscientific Model Development, v. 6, no. 3, p. 687–720. [Also available at https://doi.org/10.5194/gmd-6-687-2013.]
Briley, L.J., Rood, R.B., and Notaro, M., 2021, Large lakes in climate models—A Great Lakes case study on the usability of CMIP5: Journal of Great Lakes Research, v. 47, no. 2, p. 405–418. [Also available at https://doi.org/10.1016/j.jglr.2021.01.010.]
Bruch, R.M., Haxton, T.J., Koenigs, R., Welsh, A., and Kerr, S.J., 2016, Status of Lake Sturgeon (Acipenser fulvescens Rafinesque 1817) in North America: Journal of Applied Ichthyology, v. 32, no. S1 (special issue), p. 162–190. [Also available at https://doi.org/10.1111/jai.13240.]
Buchinger, T.J., Hondorp, D.W., and Krueger, C.C., 2022, Local diversity in phenological responses of migratory lake sturgeon to warm winters: Oikos, v. 2022, no. 6, article e08977, 6 p., accessed April 15, 2023, at https://doi.org/10.1111/oik.08977.
Burn, D.H., and Whitfield, P.H., 2023, Climate related changes to flood regimes show an increasing rainfall influence: Journal of Hydrology, v. 617, article 129075, 13 p., accessed January 16, 2023, at https://doi.org/10.1016/j.jhydrol.2023.129075.
Byun, K., Chiu, C.-M., and Hamlet, A.F., 2019, Effects of 21st century climate change on seasonal flow regimes and hydrologic extremes over the Midwest and Great Lakes region of the US: Science of the Total Environment, v. 650, no. 13, p. 1261–1277. [Also available at https://doi.org/10.1016/j.scitotenv.2018.09.063.]
Cech, J.J., Jr., and Doroshov, S.I., 2004, Environmental requirements, preferences, and tolerance limits of North American sturgeons, chap. 3 of LeBreton, G.T.O., Beamish, F.W.H., and McKinley, R.S., eds., Sturgeons and paddlefish of North America: Dordrecht, Kluwer Academic Publishers, p. 73–86. [Also available at https://doi.org/10.1007/1-4020-2833-4_4.]
Chapra, S.C., Boehlert, B., Fant, C., Bierman, V.J., Jr., Henderson, J., Mills, D., Mas, D.M.L., Rennels, L., Jantarasami, L., Martinich, J., Strzepek, K.M., and Paerl, H.W., 2017, Climate change impacts on harmful algal blooms in U.S. freshwaters—A screening-level assessment: Environmental Science & Technology, v. 51, no. 16, p. 8933–8943. [Also available at https://doi.org/10.1021/acs.est.7b01498.]
Chu, C., and Jones, N.E., 2011, Spatial variability of thermal regimes and other environmental determinants of stream fish communities in the Great Lakes Basin, Ontario, Canada: River Research and Applications, v. 27, no. 5, p. 646–662. [Also available at https://doi.org/10.1002/rra.1386.]
Collins, W.J., Bellouin, N., Doutriaux-Boucher, M., Gedney, N., Halloran, P., Hinton, T., Hughes, J., Jones, C.D., Joshi, M., Liddicoat, S., Martin, G., O’Connor, F., Rae, J., Senior, C., Sitch, S., Totterdell, I., Wiltshire, A., and Woodward, S., 2011, Development and evaluation of an Earth-System model—HadGEM2: Geoscientific Model Development, v. 4, no. 4, p. 1051–1075. [Also available at https://doi.org/10.5194/gmd-4-1051-2011.]
Committee on the Status of Endangered Wildlife in Canada, 2006, COSEWIS annual report: Committee on the Status of Endangered Wildlife in Canada [variously paged]. [Also available at https://www.canada.ca/en/environment-climate-change/services/species-risk-public-registry/cosewic-annual-reports/cosewic-annual-report-2006.html.]
Constantz, J., 1998, Interaction between stream temperature, streamflow, and groundwater exchanges in alpine streams: Water Resources Research, v. 34, no. 7, p. 1609–1615. [Also available at https://doi.org/10.1029/98WR00998.]
Dammerman, K.J., Webb, M.A.H., and Scribner, K.T., 2019, Riverine characteristics and adult demography influence female lake sturgeon (Acipenser fulvescens) spawning behavior, reproductive success, and ovarian quality: Canadian Journal of Fisheries and Aquatic Sciences, v. 76, no. 7, p. 1147–1160. [Also available at https://doi.org/10.1139/cjfas-2018-0141.]
Deacu, D., Fortin, V., Klyszejko, E., Spence, C., and Blanken, P.D., 2012, Predicting the net basin supply to the Great Lakes with a hydrometeorological model: Journal of Hydrometeorology, v. 13, no. 6, p. 1739–1759. [Also available at https://doi.org/10.1175/JHM-D-11-0151.1.]
Dhakal, N., and Palmer, R.N., 2020, Changing river flood timing in the northeastern and Upper Midwest United States—Weakening of seasonality over time?: Water, v. 12, no. 7, article 1951, 14 p., accessed April 27, 2023, at https://doi.org/10.3390/w12071951.
Diffenbaugh, N.S., Scherer, M., and Trapp, R.J., 2013, Robust increases in severe thunderstorm environments in response to greenhouse forcing: Proceedings of the National Academy of Sciences of the United States of America, v. 110, no. 41, p. 16361–16366. [Also available at https://doi.org/10.1073/pnas.1307758110.]
Dobiesz, N.E., and Lester, N.P., 2009, Changes in mid-summer water temperature and clarity across the Great Lakes between 1968 and 2002: Journal of Great Lakes Research, v. 35, no. 3, p. 371–384. [Also available at https://doi.org/10.1016/j.jglr.2009.05.002.]
Dudley, R.W., Hodgkins, G.A., McHale, M.R., Kolian, M.J., and Renard, B., 2017, Trends in snowmelt-related streamflow timing in the conterminous United States: Journal of Hydrology, v. 547, no. 4, p. 208–221. [Also available at https://doi.org/10.1016/j.jhydrol.2017.01.051.]
Dumont, P., Fortin, R., Desjardins, G., and Bernard, M., 1987, Biology and exploitation of lake sturgeon (Acipenser fulvescens) in the Quebec waters of the Saint-Laurent River, in Oliver, C.H., ed., Proceedings of a workshop on the lake sturgeon (Acipenser fulvescens): Toronto, Ontario, Ontario Ministry of Natural Resources, Ontario Fisheries Technical Report Series no. 23, p. 57–76.
Dunne, J.P., John, J.G., Adcroft, A.J., Griffies, S.M., Hallberg, R.W., Shevliakova, E., Stouffer, R.J., Cooke, W., Dunne, K.A., Harrison, M.J., Krasting, J.P., Malyshev, S.L., Milly, P.C.D., Phillipps, P.J., Sentman, L.T., Samuels, B.L., Spelman, M.J., Winton, M., Wittenberg, A.T., and Zadeh, N., 2012, GFDL’s ESM2 global coupled climate–carbon Earth system models, part I—Physical formulation and baseline simulation characteristics: Journal of Climate, v. 25, no. 19, p. 6646–6665. [Also available at https://doi.org/10.1175/JCLI-D-11-00560.1.]
Dyer, J.L., and Mote, T.L., 2006, Spatial variability and trends in observed snow depth over North America: Geophysical Research Letters, v. 33, no. 16, article L16503, 6 p., accessed April 15, 2023, at https://doi.org/10.1029/2006GL027258.
Easterling, D.R., Kunkel, K.E., Arnold, J.R., Knutson, T., LeGrande, A.N., Leung, L.R., Vose, R.S., Waliser, D.E., and Wehner, M.F., 2017, Precipitation change in the United States, chap. 7 of Wuebbles, D.J., Fahey, D.W., Hibbard, K.A., Dokken, D.J., Stewart, B.C., and Maycock, T.K., eds., Climate Science Special Report—Fourth National Climate Assessment, volume I: Washington, D.C., U.S. Global Change Research Program, p. 207–230, accessed May 16, 2023, at https://science2017.globalchange.gov/chapter/7/.
Ecclestone, A., Haxton, T.J., Pratt, T.C., Wilson, C.C., and Whillans, T., 2020, Seasonal use of two unregulated Lake Superior tributaries by lake sturgeon: Journal of Great Lakes Research, v. 46, no. 5, p. 1369–1381. [Also available at https://doi.org/10.1016/j.jglr.2020.06.008.]
Escobar, L.E., Mallez, S., McCartney, M., Lee, C., Zielinski, D.P., Ghosal, R., Bajer, P.G., Wagner, C., Nash, B., Tomamichel, M., Venturelli, P., Mathai, P.P., Kokotovich, A., Escobar-Dodero, J., and Phelps, N.B.D., 2018, Aquatic invasive species in the Great Lakes region—An overview: Reviews in Fisheries Science & Aquaculture, v. 26, no. 1, p. 121–138. [Also available at https://doi.org/10.1080/23308249.2017.1363715.]
Feng, Z., Leung, L.R., Hagos, S., Houze, R.A., Burleyson, C.D., and Balaguru, K., 2016, More frequent intense and long-lived storms dominate the springtime trend in central US rainfall: Nature Communications, v. 7, no. 1, article 13429, 8 p., accessed April 20, 2023, at https://doi.org/10.1038/ncomms13429.
Ficklin, D.L., Stewart, I.T., and Maurer, E.P., 2013, Effects of climate change on stream temperature, dissolved oxygen, and sediment concentration in the Sierra Nevada in California: Water Resources Research, v. 49, no. 5, p. 2765–2782. [Also available at https://doi.org/10.1002/wrcr.20248.]
Forsythe, P.S., Crossman, J.A., Bello, N.M., Baker, E.A., and Scribner, K.T., 2011, Individual-based analyses reveal high repeatability in timing and location of reproduction in lake sturgeon (Acipenser fulvescens): Canadian Journal of Fisheries and Aquatic Sciences, v. 69, no. 1, p. 60–72. [Also available at https://doi.org/10.1139/f2011-132.]
Gent, P.R., Danabasoglu, G., Donner, L.J., Holland, M.M., Hunke, E.C., Jayne, S.R., Lawrence, D.M., Neale, R.B., Rasch, P.J., Vertenstein, M., Worley, P.H., Yang, Z.-L., and Zhang, M., 2011, The Community Climate System Model version 4: Journal of Climate, v. 24, no. 19, p. 4973–4991. [Also available at https://doi.org/10.1175/2011JCLI4083.1.]
GLIFWC Climate Change Team, 2023, Aanji-bimaadiziimagak o’ow aki: Odanah, Wis., Great Lakes Indian Fish and Wildlife Commission, 332 p., accessed June 21, 2023, at https://data.glifwc.org/download/archive.bio/Aanji-bimaadiziimagak_o_ow_aki_digital_02212023.pdf.
Gronewold, A.D., Bruxer, J., Durnford, D., Smith, J.P., Clites, A.H., Seglenieks, F., Qian, S.S., Hunter, T.S., and Fortin, V., 2016, Hydrological drivers of record-setting water level rise on Earth’s largest lake system: Water Resources Research, v. 52, no. 5, p. 4026–4042. [Also available at https://doi.org/10.1002/2015WR018209.]
Gronewold, A.D., and Rood, R.B., 2019, Recent water level changes across Earth’s largest lake system and implications for future variability: Journal of Great Lakes Research, v. 45, no. 1, p. 1–3. [Also available at https://doi.org/10.1016/j.jglr.2018.10.012.]
Haxton, T.J., Sulak, K., and Hildebrand, L., 2016, Status of scientific knowledge of North American sturgeon: Journal of Applied Ichthyology, v. 32, no. S1 (special issue), p. 5–10. [Also available at https://doi.org/10.1111/jai.13235.]
Hoerling, M., Eischeid, J., Perlwitz, J., Quan, X.-W., Wolter, K., and Cheng, L., 2016, Characterizing recent trends in U.S. heavy precipitation: Journal of Climate, v. 29, no. 7, p. 2313–2332. [Also available at https://doi.org/10.1175/JCLI-D-15-0441.1.]
Hupfeld, R.N., Phelps, Q.E., Flammang, M.K., and Whitledge, G.W., 2015, Assessment of the effects of high summer water temperatures on shovelnose sturgeon and potential implications of climate change: River Research and Applications, v. 31, no. 9, p. 1195–1201. [Also available at https://doi.org/10.1002/rra.2806.]
Jackson, J.R., VanDeValk, A.J., Brooking, T.E., vanKeeken, O.A., and Rudstam, L.G., 2002, Growth and feeding dynamics of lake sturgeon, Acipenser fulvescens, in Oneida Lake, New York—Results from the first five years of a restoration program: Journal of Applied Ichthyology, v. 18, nos. 4–6, p. 439–443. [Also available at https://doi.org/10.1046/j.1439-0426.2002.00394.x.]
Ji, D., Wang, L., Feng, J., Wu, Q., Cheng, H., Zhang, Q., Yang, J., Dong, W., Dai, Y., Gong, D., Zhang, R.-H., Wang, X., Liu, J., Moore, J.C., Chen, D., and Zhou, M., 2014, Description and basic evaluation of Beijing Normal University Earth System Model (BNU–ESM) version 1: Geoscientific Model Development, v. 7, no. 5, p. 2039–2064. [Also available at https://doi.org/10.5194/gmd-7-2039-2014.]
Kampa, J., Hatzenbeler, G., and Jennings, M., 2014, Status and management of lake sturgeon (Acipenser fulvescens Rafinesque, 1817) in the upper St. Croix River and Namekagon River, Wisconsin, USA: Journal of Applied Ichthyology, v. 30, no. 6 (special issue), p. 1387–1392. [Also available at https://doi.org/10.1111/jai.12541.]
Kayastha, M.B., Ye, X., Huang, C., and Xue, P., 2022, Future rise of the Great Lakes water levels under climate change: Journal of Hydrology, v. 612, no. 8, article 128205, 13 p., accessed April 15, 2023, at https://doi.org/10.1016/j.jhydrol.2022.128205.
Kornis, M.S., Mercado-Silva, N., and Vander Zanden, M.J., 2012, Twenty years of invasion—A review of round goby Neogobius melanostomus biology, spread and ecological implications: Journal of Fish Biology, v. 80, no. 2, p. 235–285. [Also available at https://doi.org/10.1111/j.1095-8649.2011.03157.x.]
Lanzante, J.R., Adams‐Smith, D., Dixon, K.W., Nath, M., and Whitlock, C.E., 2020, Evaluation of some distributional downscaling methods as applied to daily maximum temperature with emphasis on extremes: International Journal of Climatology, v. 40, no. 3, p. 1571–1585. [Also available at https://doi.org/10.1002/joc.6288.]
Li, D., Lettenmaier, D.P., Margulis, S.A., and Andreadis, K., 2019, The role of rain‐on‐snow in flooding over the conterminous United States: Water Resources Research, v. 55, no. 11, p. 8492–8513. [Also available at https://doi.org/10.1029/2019WR024950.]
Lisi, P.J., Schindler, D.E., Cline, T.J., Scheuerell, M.D., and Walsh, P.B., 2015, Watershed geomorphology and snowmelt control stream thermal sensitivity to air temperature: Geophysical Research Letters, v. 42, no. 9, p. 3380–3388. [Also available at https://doi.org/10.1002/2015GL064083.]
Lofgren, B.M., Hunter, T.S., and Wilbarger, J., 2011, Effects of using air temperature as a proxy for potential evapotranspiration in climate change scenarios of Great Lakes basin hydrology: Journal of Great Lakes Research, v. 37, no. 4, p. 744–752. [Also available at https://doi.org/10.1016/j.jglr.2011.09.006.]
Lofgren, B.M., and Rouhana, J., 2016, Physically plausible methods for projecting changes in Great Lakes water levels under climate change scenarios: Journal of Hydrometeorology, v. 17, no. 8, p. 2209–2223. [Also available at https://doi.org/10.1175/JHM-D-15-0220.1.]
Lopez, H., West, R., Dong, S., Goni, G., Kirtman, B., Lee, S.-K., and Atlas, R., 2018, Early emergence of anthropogenically forced heat waves in the western United States and Great Lakes: Nature Climate Change, v. 8, no. 5, p. 414–420. [Also available at https://doi.org/10.1038/s41558-018-0116-y.]
Lyons, J., and Stewart, J.S., 2014, Predicted effects of future climate warming on thermal habitat suitability for Lake Sturgeon (Acipenser fulvescens, Rafinesque, 1817) in rivers in Wisconsin, USA: Journal of Applied Ichthyology, v. 30, no. 6 (special issue), p. 1508–1513. [Also available at https://doi.org/10.1111/jai.12543.]
Magnuson, J.J., Webster, K.E., Assel, R.A., Bowser, C.J., Dillon, P.J., Eaton, J.G., Evans, H.E., Fee, E.J., Hall, R.I., Mortsch, L.R., Schindler, D.W., and Quinn, F.H., 1997, Potential effects of climate changes on aquatic systems—Laurentian Great Lakes and Precambrian Shield region: Hydrological Processes, v. 11, no. 8, p. 825–871. [Also available at https://doi.org/10.1002/(SICI)1099-1085(19970630)11:8%3C825::AID-HYP509%3E3.0.CO;2-G.]
Mailhot, E., Music, B., Nadeau, D.F., Frigon, A., and Turcotte, R., 2019, Assessment of the Laurentian Great Lakes’ hydrological conditions in a changing climate: Climatic Change, v. 157, no. 2, p. 243–259. [Also available at https://doi.org/10.1007/s10584-019-02530-6.]
Mallakpour, I., and Villarini, G., 2015, The changing nature of flooding across the central United States: Nature Climate Change, v. 5, no. 3, p. 250–254. [Also available at https://doi.org/10.1038/nclimate2516.]
Mallard, M.S., Nolte, C.G., Bullock, O.R., Spero, T.L., and Gula, J., 2014, Using a coupled lake model with WRF for dynamical downscaling: Journal of Geophysical Research—Atmospheres, v. 119, no. 12, p. 7193–7208. [Also available at https://doi.org/10.1002/2014JD021785.]
Mallard, M.S., Nolte, C.G., Spero, T.L., Bullock, O.R., Alapaty, K., Herwehe, J.A., Gula, J., and Bowden, J.H., 2015, Technical challenges and solutions in representing lakes when using WRF in downscaling applications: Geoscientific Model Development, v. 8, no. 4, p. 1085–1096. [Also available at https://doi.org/10.5194/gmd-8-1085-2015.]
Marti, O., Braconnot, P., Dufresne, J.-L., Bellier, J., Benshila, R., Bony, S., Brockmann, P., Cadule, P., Caubel, A., Codron, F., de Noblet, N., Denvil, S., Fairhead, L., Fichefet, T., Foujols, M.-A., Friedlingstein, P., Goosse, H., Grandpeix, J.-Y., Guilyardi, E., Hourdin, F., Idelkadi, A., Kageyama, M., Krinner, G., Lévy, C., Madec, G., Mignot, J., Musat, I., Swingedouw, D., and Talandier, C., 2010, Key features of the IPSL ocean atmosphere model and its sensitivity to atmospheric resolution: Climate Dynamics, v. 34, no. 1, p. 1–26. [Also available at https://doi.org/10.1007/s00382-009-0640-6.]
Mason, L.A., Riseng, C.M., Gronewold, A.D., Rutherford, E.S., Wang, J., Clites, A., Smith, S.D.P., and McIntyre, P.B., 2016, Fine-scale spatial variation in ice cover and surface temperature trends across the surface of the Laurentian Great Lakes: Climatic Change, v. 138, nos. 1–2, p. 71–83. [Also available at https://doi.org/10.1007/s10584-016-1721-2.]
McCabe, D.J., Beekey, M, Mazloff, A., and Marsden, I.E., 2006, Negative effect of zebra mussels on foraging and habitat use by lake sturgeon (Acipenser fulvescens): Biology Faculty Publications no. 64, 8 p. [Also available at https://digitalcommons.sacredheart.edu/bio_fac/64.]
McCabe, G.J., and Wolock, D.M., 2014, Spatial and temporal patterns in conterminous United States streamflow characteristics: Geophysical Research Letters, v. 41, no. 19, p. 6889–6897. [Also available at https://doi.org/10.1002/2014GL061980.]
Mearns, L., McGinnis, S., Korytina, D., Arritt, R., Biner, S., Bukovsky, M., Chang, H.-I., Christensen, O., Herzmann, D., Jiao, Y., Kharin, S., Lazare, M., Nikulin, G., Qian, M., Scinocca, J., Winger, K., Castro, C., Frigon, A., and Gutowski, W., 2017, The NA-CORDEX dataset, version 1.0: Boulder, Colo., NCAR Climate Data Gateway digital data, accessed May 16, 2023, at https://doi.org/10.5065/D6SJ1JCH.
Mo, K.C., and Lettenmaier, D.P., 2020, Prediction of flash droughts over the United States: Journal of Hydrometeorology, v. 21, no. 8, p. 1793–1810. [Also available at https://doi.org/10.1175/JHM-D-19-0221.1.]
Moore, M.J., Paukert, C.P., and Moore, T.L., 2021, Effects of latitude, season, and temperature on Lake Sturgeon movement: North American Journal of Fisheries Management, v. 41, no. 4, p. 916–928. [Also available at https://doi.org/10.1002/nafm.10416.]
Moore, M.J., Paukert, C.P., Owens, S.P., and Moore, T.L., 2022, Habitat selection in a southern Lake Sturgeon population—Implications of temporal, spatial, and ontogenetic variation for restoration: Restoration Ecology, v. 30, no. 7, article e13602, 15 p., accessed May 15, 2023, at https://doi.org/10.1111/rec.13602.
Mulvaney, K.K., Foley, C.J., Höök, T.O., McNie, E.C., and Prokopy, L.S., 2014, Identifying useful climate change information needs of Great Lakes fishery managers: Journal of Great Lakes Research, v. 40, no. 3, p. 590–598. [Also available at https://doi.org/10.1016/j.jglr.2014.06.002.]
NatureServe, 2022, 2022 annual report: NatureServe, 7 p. [Also available at https://www.natureserve.org/sites/default/files/2023-06/NatureServe%20Annual%20Report%202022_pages_2.pdf.]
Norton, R.K., Buckman, S., Meadows, G.A., and Rable, Z., 2019, Using simple, decision-centered, scenario-based planning to improve local coastal management: Journal of the American Planning Association, v. 85, no. 4, p. 405–423. [Also available at https://doi.org/10.1080/01944363.2019.1627237.]
Notaro, M., Bennington, V., and Lofgren, B., 2015a, Dynamical downscaling-based projections of Great Lakes water levels: Journal of Climate, v. 28, no. 24, p. 9721–9745. [Also available at https://doi.org/10.1175/JCLI-D-14-00847.1.]
Notaro, M., Bennington, V., and Vavrus, S., 2015b, Dynamically downscaled projections of lake-effect snow in the Great Lakes basin: Journal of Climate, v. 28, no. 4, p. 1661–1684. [Also available at https://doi.org/10.1175/JCLI-D-14-00467.1.]
Notaro, M., Holman, K., Zarrin, A., Fluck, E., Vavrus, S., and Bennington, V., 2013, Influence of the Laurentian Great Lakes on regional climate: Journal of Climate, v. 26, no. 3, p. 789–804. [Also available at https://doi.org/10.1175/JCLI-D-12-00140.1.]
Notaro, M., Jorns, J., and Briley, L., 2022, Representation of lake-atmosphere interactions and lake-effect snowfall in the Laurentian Great Lakes basin among HighResMIP global climate models: Journal of the Atmospheric Sciences, v. 79, no. 5, p. 1325–1347. [Also available at https://doi.org/10.1175/JAS-D-21-0249.1.]
Notaro, M., Lorenz, D.J., Vimont, D., Vavrus, S., Kucharik, C., and Franz, K., 2011, 21st century Wisconsin snow projections based on an operational snow model driven by statistically downscaled climate data: International Journal of Climatology, v. 31, no. 11, p. 1615–1633. [Also available at https://doi.org/10.1002/joc.2179.]
Notaro, M., Zhong, Y., Xue, P., Peters-Lidard, C., Cruz, C., Kemp, E., Kristovich, D., Kulie, M., Wang, J., Huang, C., and Vavrus, S.J., 2021, Cold season performance of the NU-WRF regional climate model in the Great Lakes region: Journal of Hydrometeorology, v. 22, no. 9, p. 2423–2454. [Also available at https://doi.org/10.1175/JHM-D-21-0025.1.]
O’Reilly, C.M., Sharma, S., Gray, D.K., Hampton, S.E., Read, J.S., Rowley, R.J., Schneider, P., Lenters, J.D., McIntyre, P.B., Kraemer, B.M., Weyhenmeyer, G.A., Straile, D., Dong, B., Adrian, R., Allan, M.G., Anneville, O., Arvola, L., Austin, J., Bailey, J.L., Baron, J.S., Brookes, J.D., de Eyto, E., Dokulil, M.T., Hamilton, D.P., Havens, K., Hetherington, A.L., Higgins, S.N., Hook, S., Izmest’eva, L.R., Joehnk, K.D., Kangur, K., Kasprzak, P., Kumagai, M., Kuusisto, E., Leshkevich, G., Livingstone, D.M., MacIntyre, S., May, L., Melack, J.M., Mueller-Navarra, D.C., Naumenko, M., Noges, P., Noges, T., North, R.P., Plisnier, P.-D., Rigosi, A., Rimmer, A., Rogora, M., Rudstam, L.G., Rusak, J.A., Salmaso, N., Samal, N.R., Schindler, D.E., Schladow, S.G., Schmid, M., Schmidt, S.R., Silow, E., Soylu, M.E., Teubner, K., Verburg, P., Voutilainen, A., Watkinson, A., Williamson, C.E., and Zhang, G., 2015, Rapid and highly variable warming of lake surface waters around the globe: Geophysical Research Letters, v. 42, no. 24, p. 10773–10781. [Also available at https://doi.org/10.1002/2015GL066235.]
Paradis, Y., Bernatchez, S., Valiquette, É., Mingelbier, M., Hatin, D., Brodeur, P., Paquin, É., Côté, C., L’Italien, L., Calvé, T., Hamel, J.-P., Bélanger, M., and Haxton, T.J., 2022, A review of lake sturgeon Acipenser fulvescens spawning sites in the Lower St. Lawrence and Ottawa river systems: Journal of Great Lakes Research, v. 48, no. 4, p. 857–864. [Also available at https://doi.org/10.1016/j.jglr.2022.05.011.]
Rahel, F.J., and Olden, J.D., 2008, Assessing the effects of climate change on aquatic invasive species: Conservation Biology, v. 22, no. 3, p. 521–533. [Also available at https://doi.org/10.1111/j.1523-1739.2008.00950.x.]
Rotstayn, L.D., Collier, M.A., Dix, M.R., Feng, Y., Gordon, H.B., O’Farrell, S.P., Smith, I.N., and Syktus, J., 2010, Improved simulation of Australian climate and ENSO-related rainfall variability in a global climate model with an interactive aerosol treatment: International Journal of Climatology, v. 30, no. 7, p. 1067–1088. [Also available at https://doi.org/10.1002/joc.1952.]
Schloesser, J.T., and Quinlan, H.R., 2019, Population status and demographics of Lake Sturgeon in the Bad and White rivers, Wisconsin: Journal of Fish and Wildlife Management, v. 10, no. 2, p. 442–457. [Also available at https://doi.org/10.3996/022019-JFWM-005.]
Seglenieks, F., and Temgoua, A., 2022, Future water levels of the Great Lakes under 1.5°C to 3°C warmer climates: Journal of Great Lakes Research, v. 48, no. 4, p. 865–875. [Also available at https://doi.org/10.1016/j.jglr.2022.05.012.]
Sharma, A., Hamlet, A.F., Fernando, H.J.S., Catlett, C.E., Horton, D.E., Kotamarthi, V.R., Kristovich, D.A.R., Packman, A.I., Tank, J.L., and Wuebbles, D.J., 2018, The need for an integrated land-lake-atmosphere modeling system, exemplified by North America’s Great Lakes region: Earth’s Future, v. 6, no. 10, p. 1366–1379. [Also available at https://doi.org/10.1029/2018EF000870.]
Shinohara, R., Matsuzaki, S.S., Watanabe, M., Nakagawa, M., Yoshida, H., and Kohzu, A., 2023, Heat waves can cause hypoxia in shallow lakes: Geophysical Research Letters, v. 50, no. 8, article e2023GL102967, 10 p., accessed April 22, 2023, at https://doi.org/10.1029/2023GL102967.
Shrestha, N.K., Seglenieks, F., Temgoua, A.G.T., and Dehghan, A., 2022, The impacts of climate change on land hydroclimatology of the Laurentian Great Lakes basin: Frontiers in Water, v. 4, article 801134, 22 p., accessed July 13, 2022, at https://doi.org/10.3389/frwa.2022.801134.
Spence, C., Blanken, P.D., Lenters, J.D., and Hedstrom, N., 2013, The importance of spring and autumn atmospheric conditions for the evaporation regime of Lake Superior: Journal of Hydrometeorology, v. 14, no. 5, p. 1647–1658. [Also available at https://doi.org/10.1175/JHM-D-12-0170.1.]
Stults, M., Petersen, S., Bell, J., Baule, W., Nasser, E., Gibbons, E., and Fougerat, M., 2016, Climate change vulnerability assessment and adaptation plan—1854 Ceded Territory including the Bois Forte, Fond du Lac, and Grand Portage Reservations: Duluth, Minn., 1854 Treaty Authority, 146 p., accessed June 22, 2023, at https://www.1854treatyauthority.org/images/ClimateAdaptationPlan_Final-July_2016-optimized(1).pdf.
Taylor, K.E., Stouffer, R.J., and Meehl, G.A., 2012, An overview of CMIP5 and the experiment design: Bulletin of the American Meteorological Society, v. 93, no. 4, p. 485–498. [Also available at https://doi.org/10.1175/BAMS-D-11-00094.1.]
Tellier, J.M., Kalejs, N.I., Leonhardt, B.S., Cannon, D., Hӧӧk, T.O., and Collingsworth, P.D., 2022, Widespread prevalence of hypoxia and the classification of hypoxic conditions in the Laurentian Great Lakes: Journal of Great Lakes Research, v. 48, no. 1, p. 13–23. [Also available at https://doi.org/10.1016/j.jglr.2021.11.004.]
Theuerkauf, E.J., and Braun, K.N., 2021, Rapid water level rise drives unprecedented coastal habitat loss along the Great Lakes of North America: Journal of Great Lakes Research, v. 47, no. 4, p. 945–954. [Also available at https://doi.org/10.1016/j.jglr.2021.05.004.]
Thomas, M.V., and Haas, R.C., 2002, Abundance, age structure, and spatial distribution of lake sturgeon, Acipenser fulvescens, in the St Clair System: Journal of Applied Ichthyology, v. 18, nos. 4–6, p. 495–501. [Also available at https://doi.org/10.1046/j.1439-0426.2002.00387.x.]
Van Cleave, K., Lenters, J.D., Wang, J., and Verhamme, E.M., 2014, A regime shift in Lake Superior ice cover, evaporation, and water temperature following the warm El Niño winter of 1997–1998: Limnology and Oceanography, v. 59, no. 6, p. 1889–1898. [Also available at https://doi.org/10.4319/lo.2014.59.6.1889.]
van der Lee, A.S., Gardner, W.M., O’Connor, L.M., Pratt, T.C., and Koops, M.A., 2022, Lake Sturgeon (Acipenser fulvescens) in Goulais Bay, Lake Superior—Cohort strength determinants and population viability: Journal of Great Lakes Research, v. 48, no. 5, p. 1278–1287. [Also available at https://doi.org/10.1016/j.jglr.2022.08.007.]
VanDeWeghe, A., Lin, V., Jayaram, J., and Gronewold, A.D., 2022, Changes in large lake water level dynamics in response to climate change: Frontiers in Water, v. 4, article 805143, 12 p., accessed April 15, 2023, at https://doi.org/10.3389/frwa.2022.805143.
Voldoire, A., Sanchez-Gomez, E., Salas y Mélia, D., Decharme, B., Cassou, C., Sénési, S., Valcke, S., Beau, I., Alias, A., Chevallier, M., Déqué, M., Deshayes, J., Douville, H., Fernandez, E., Madec, G., Maisonnave, E., Moine, M.-P., Planton, S., Saint-Martin, D., Szopa, S., Tyteca, S., Alkama, R., Belamari, S., Braun, A., Coquart, L., and Chauvin, F., 2013, The CNRM–CM5.1 global climate model—Description and basic evaluation: Climate Dynamics, v. 40, no. 9–10, p. 2091–2121. [Also available at https://doi.org/10.1007/s00382-011-1259-y.]
Volodin, E.M., Dianskii, N.A., and Gusev, A.V., 2010, Simulating present-day climate with the INMCM4.0 coupled model of the atmospheric and oceanic general circulations: Izvestiya—Atmospheric and Oceanic Physics, v. 46, no. 4, p. 414–431. [Also available at https://doi.org/10.1134/S000143381004002X.]
Vose, R.S., Easterling, D.R., Kunkel, K.E., LeGrande, A.N., and Wehner, M.F., 2017, Temperature changes in the United States, chap. 6 of Wuebbles, D.J., Fahey, D.W., Hibbard, K.A., Dokken, D.J., Stewart, B.C., and Maycock, T.K., eds., Climate Science Special Report—Fourth National Climate Assessment, volume I: Washington, D.C., U.S. Global Change Research Program, p. 185–206, accessed July 20, 2022, at https://science2017.globalchange.gov/chapter/6/.
Wang, G., Kirchhoff, C.J., Seth, A., Abatzoglou, J.T., Livneh, B., Pierce, D.W., Fomenko, L., and Ding, T., 2020, Projected changes of precipitation characteristics depend on downscaling method and training data—MACA versus LOCA using the U.S. Northeast as an example: Journal of Hydrometeorology, v. 21, no. 12, p. 2739–2758. [Also available at https://doi.org/10.1175/JHM-D-19-0275.1.]
Wang, J., Bai, X., Hu, H., Clites, A., Colton, M., and Lofgren, B., 2012, Temporal and spatial variability of Great Lakes ice cover, 1973–2010: Journal of Climate, v. 25, no. 4, p. 1318–1329. [Also available at https://doi.org/10.1175/2011JCLI4066.1.]
Wang, J., Xue, P., Pringle, W., Yang, Z., and Qian, Y., 2022, Impacts of lake surface temperature on the summer climate over the Great Lakes Region: Journal of Geophysical Research—Atmospheres, v. 127, no. 11, article e2021JD036231, 20 p., accessed April 15, 2023, at https://doi.org/10.1029/2021JD036231.
Watanabe, M., Suzuki, T., O’ishi, R., Komuro, Y., Watanabe, S., Emori, S., Takemura, T., Chikira, M., Ogura, T., Sekiguchi, M., Takata, K., Yamazaki, D., Yokohata, T., Nozawa, T., Hasumi, H., Tatebe, H., and Kimoto, M., 2010, Improved climate simulation by MIROC5—Mean states, variability, and climate sensitivity: Journal of Climate, v. 23, no. 23, p. 6312–6335. [Also available at https://doi.org/10.1175/2010JCLI3679.1.]
Welsh, A., Mohr, L., and Boase, J., 2017, Identifying conservation hotspots in non-breeding areas—A case study of Lake Sturgeon (Acipenser fulvescens) in the Great Lakes: Biodiversity and Conservation, v. 26, no. 4, p. 931–941. [Also available at https://doi.org/10.1007/s10531-016-1280-x.]
Wootten, A.M., Dixon, K.W., Adams‐Smith, D.J., and McPherson, R.A., 2021, Statistically downscaled precipitation sensitivity to gridded observation data and downscaling technique: International Journal of Climatology, v. 41, no. 2, p. 980–1001. [Also available at https://doi.org/10.1002/joc.6716.]
Xiao, C., Lofgren, B.M., Wang, J., and Chu, P.Y., 2016, Improving the lake scheme within a coupled WRF-lake model in the Laurentian Great Lakes: Journal of Advances in Modeling Earth Systems, v. 8, no. 4, p. 1969–1985. [Also available at https://doi.org/10.1002/2016MS000717.]
Xiao-Ge, X., Tong-Wen, W., and Jie, Z., 2013, Introduction of CMIP5 experiments carried out with the climate system models of Beijing Climate Center: Advances in Climate Change Research, v. 4, no. 1, p. 41–49. [Also available at https://doi.org/10.3724/SP.J.1248.2013.041.]
Xie, F., Erler, A.R., Chandan, D., and Peltier, W.R., 2021, Great Lakes Basin heat waves—An analysis of their increasing probability of occurrence under global warming: Frontiers in Water, v. 3, article 782265, 21 p., accessed April 15, 2023, at https://doi.org/10.3389/frwa.2021.782265.
Xue, P., Pal, J.S., Ye, X., Lenters, J.D., Huang, C., and Chu, P.Y., 2017, Improving the simulation of large lakes in regional climate modeling—Two-way lake-atmosphere coupling with a 3D hydrodynamic model of the Great Lakes: Journal of Climate, v. 30, no. 5, p. 1605–1627. [Also available at https://doi.org/10.1175/JCLI-D-16-0225.1.]
Xue, P., Ye, X., Pal, J.S., Chu, P.Y., Kayastha, M.B., and Huang, C., 2022, Climate projections over the Great Lakes region—Using two-way coupling of a regional climate model with a 3-D lake model: Geoscientific Model Development, 37 p., accessed June 29, 2022, at https://gmd.copernicus.org/preprints/gmd-2021-440/gmd-2021-440.pdf.
Yukimoto, S., Adachi, Y., Hosaka, M., Sakami, T., Yoshimura, H., Hirabara, M., Tanaka, T.Y., Shindo, E., Tsujino, H., Deushi, M., Mizuta, R., Yabu, S., Obata, A., Nakano, H., Koshiro, T., Ose, T., and Kitoh, A., 2012, A new global climate model of the Meteorological Research Institute—MRI–CGCM3—Model description and basic performance: Journal of the Meteorological Society of Japan, v. 90A, no. 0, p. 23–64. [Also available at https://doi.org/10.2151/jmsj.2012-A02.]
Zhang, H., Wang, J., Yang, T., Lofgren, B., and Chu, P., 2018, Statistical relationships between biological parameters and environmental forcings in Lake Erie, 1970s–2010s: Ann Arbor, Mich., National Oceanic and Atmospheric Administration, NOAA Technical Memorandum GLERL–173, 69 p. [Also available at https://doi.org/10.25923/6jgm-1x64.]
Zhong, Y., Notaro, M., Vavrus, S.J., and Foster, M.J., 2016, Recent accelerated warming of the Laurentian Great Lakes—Physical drivers: Limnology and Oceanography, v. 61, no. 5, p. 1762–1786. [Also available at https://doi.org/10.1002/lno.10331.]
Appendix 1. Climate Maps
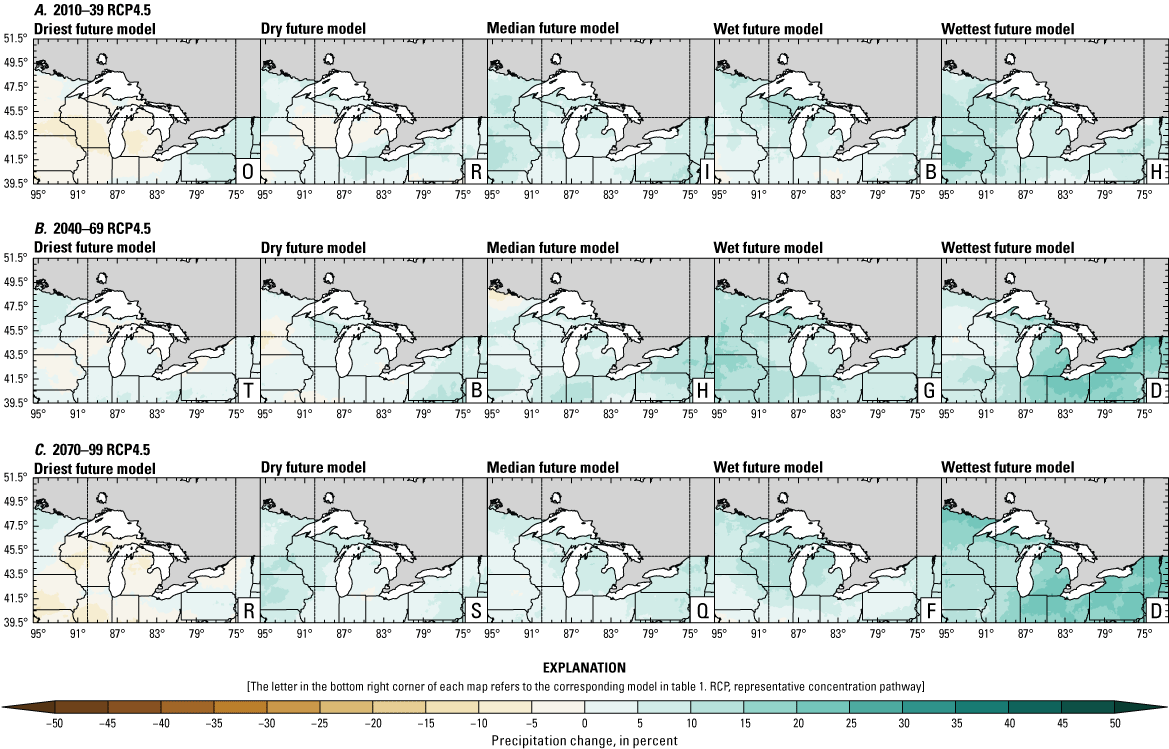

Annual mean total precipitation change (in percent) in the downscaled climate simulations under emissions scenarios for representative concentration pathway 4.5 (RCP4.5) and representative concentration pathway 8.5 (RCP8.5) from the historical period (1971–2000) to the future periods. A, early 21st century (2010–39) for RCP4.5; B, mid-21st century (2040–69) for RCP4.5; C, late 21st century (2070–99) for RCP4.5; D, early 21st century (2010–39) for RCP8.5; E, mid-21st century (2040–69) for RCP8.5; F, late 21st century (2070–99) for RCP8.5.
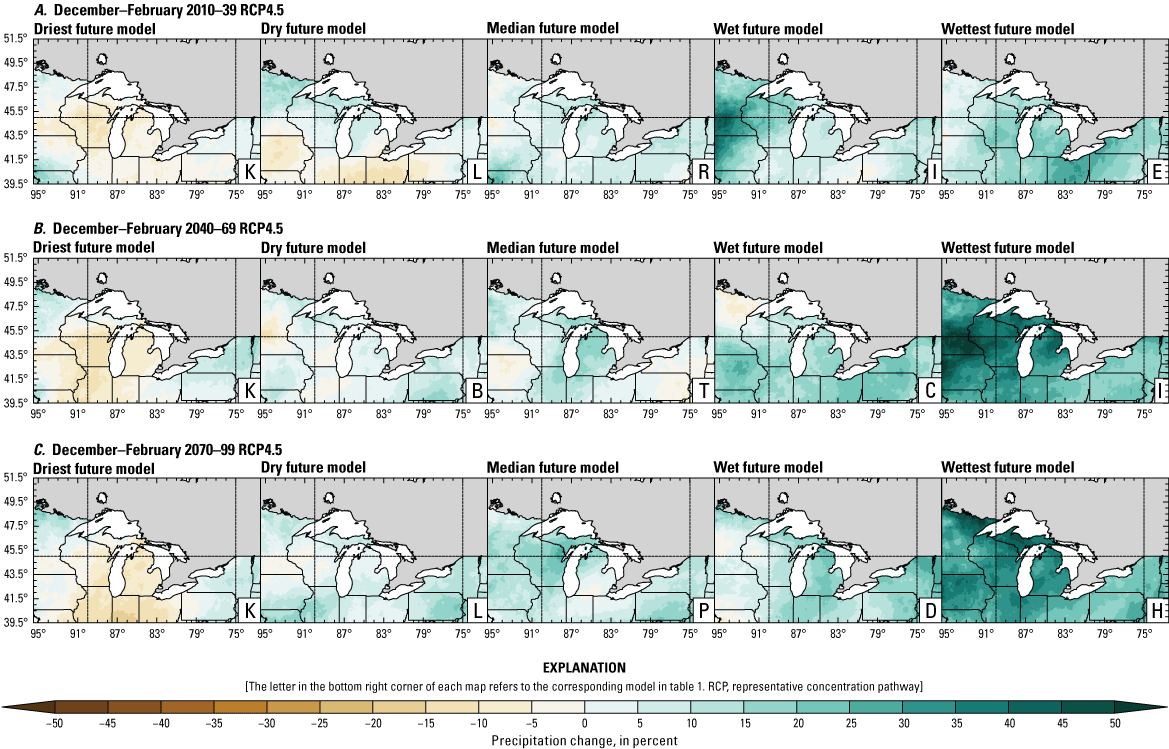
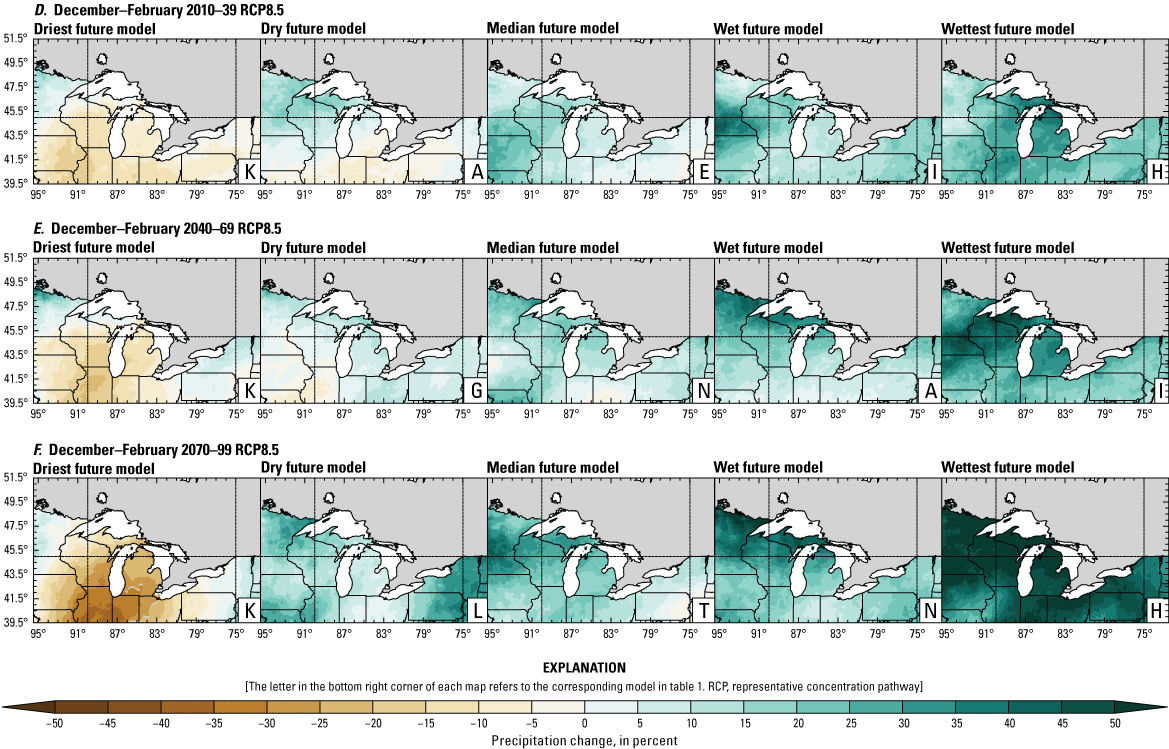
December–February mean total precipitation change (in percent) in the downscaled climate simulations under emissions scenarios for representative concentration pathway 4.5 (RCP4.5) and representative concentration pathway 8.5 (RCP8.5) from the historical period (1971–2000) to the future periods. A, early 21st century (2010–39) for RCP4.5; B, mid-21st century (2040–69) for RCP4.5; C, late 21st century (2070–99) for RCP4.5; D, early 21st century (2010–39) for RCP8.5; E, mid-21st century (2040–69) for RCP8.5; F, late 21st century (2070–99) for RCP8.5.
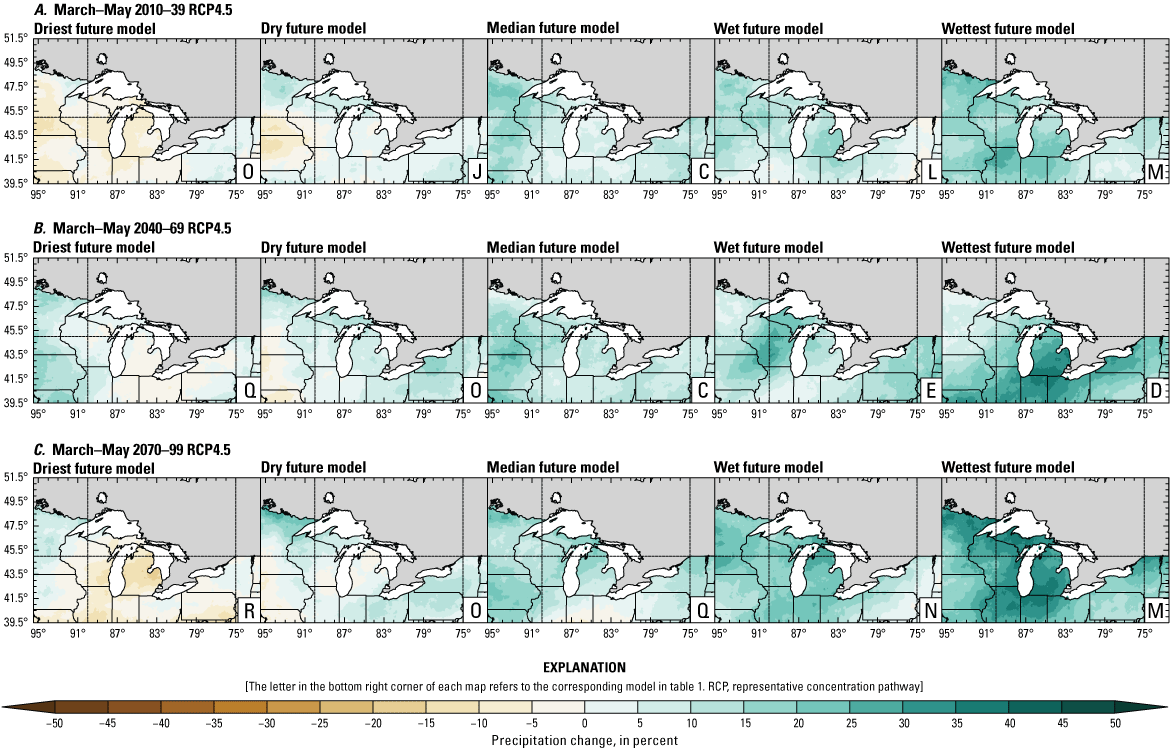
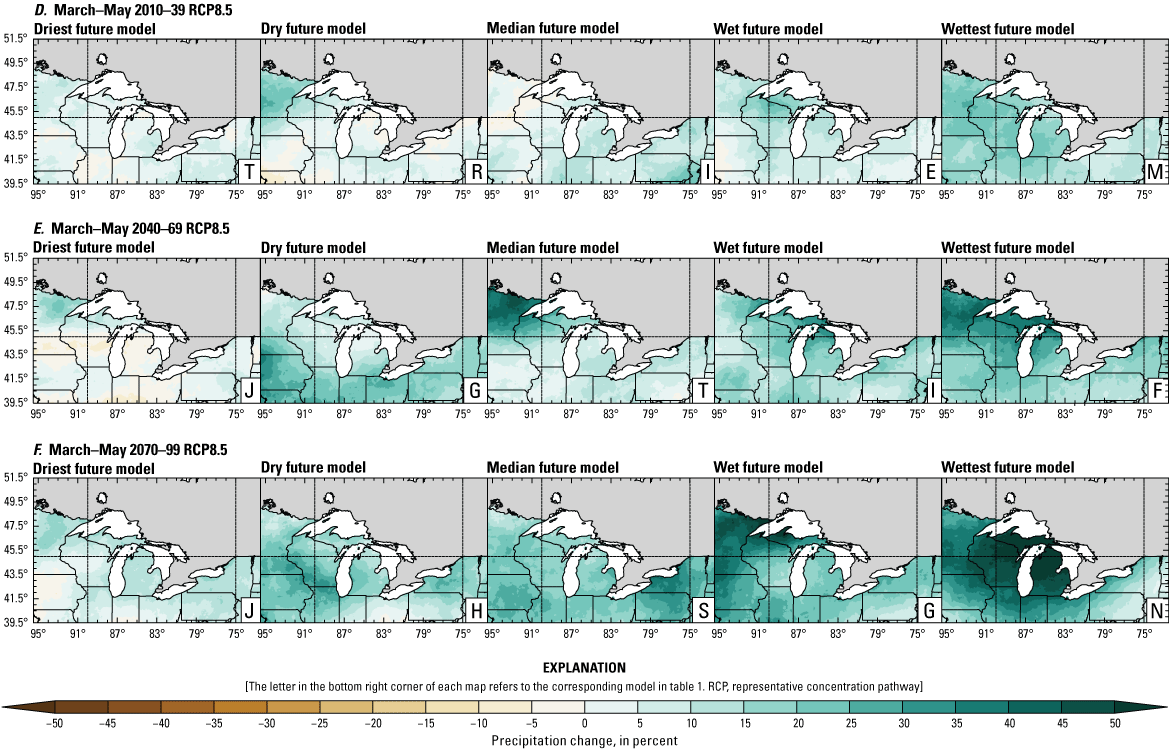
March–May mean total precipitation change (in percent) in the downscaled climate simulations under emissions scenarios for representative concentration pathway 4.5 (RCP4.5) and representative concentration pathway 8.5 (RCP8.5) from the historical period (1971–2000) to the future periods. A, early 21st century (2010–39) for RCP4.5; B, mid-21st century (2040–69) for RCP4.5; C, late 21st century (2070–99) for RCP4.5; D, early 21st century (2010–39) for RCP8.5; E, mid-21st century (2040–69) for RCP8.5; F, late 21st century (2070–99) for RCP8.5.
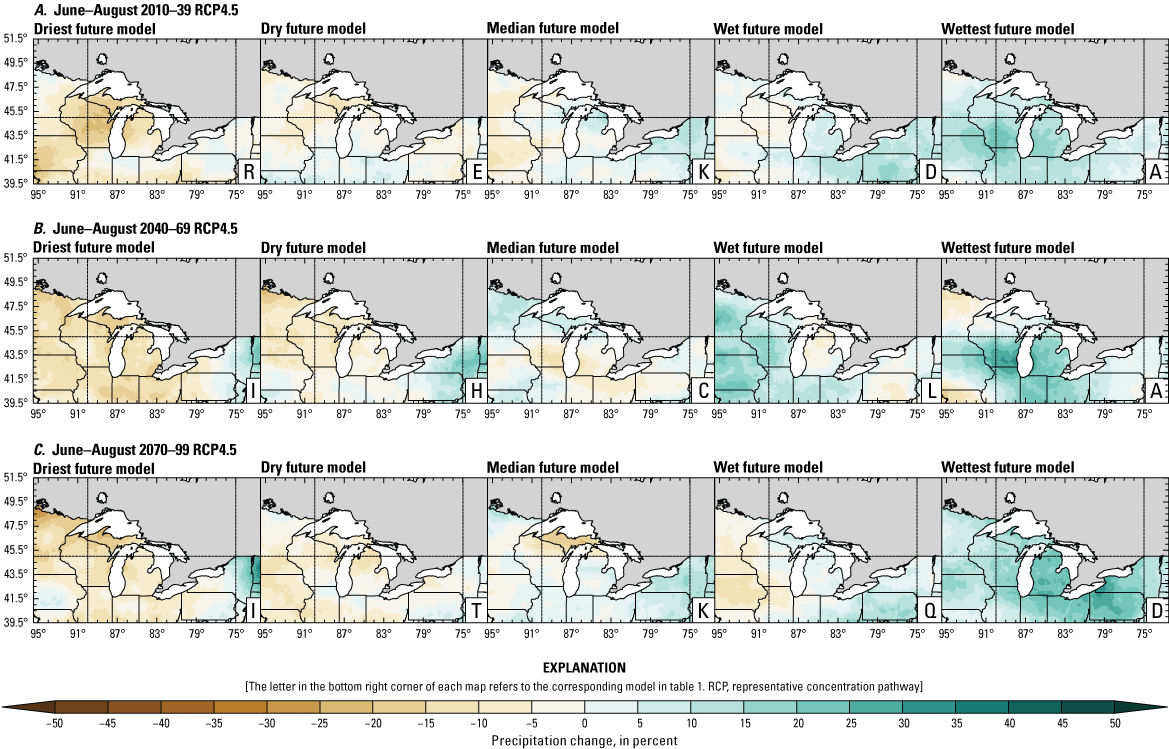

June–August mean total precipitation change (in percent) in the downscaled climate simulations under emissions scenarios for representative concentration pathway 4.5 (RCP4.5) and representative concentration pathway8.5 (RCP8.5) from the historical period (1971–2000) to the future periods. A, early 21st century (2010–39) for RCP4.5; B, mid-21st century (2040–69) for RCP4.5; C, late 21st century (2070–99) for RCP4.5; D, early 21st century (2010–39) for RCP8.5; E, mid-21st century (2040–69) for RCP8.5; F, late 21st century (2070–99) for RCP8.5.
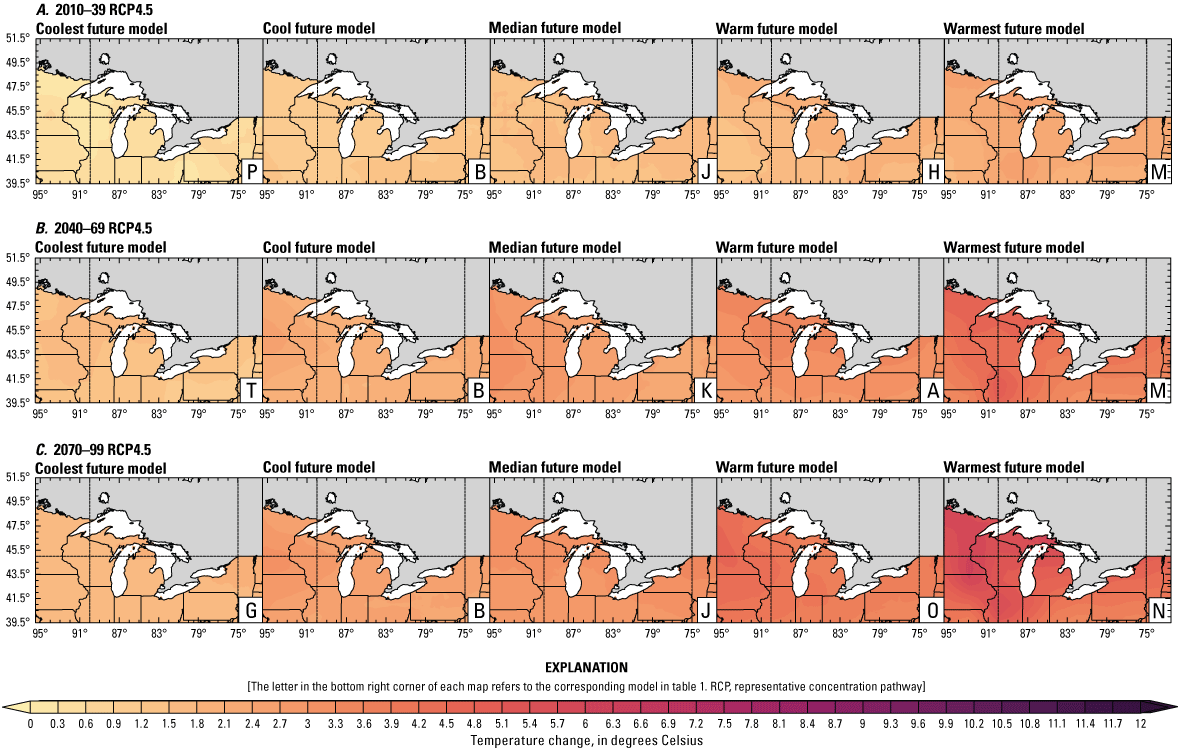
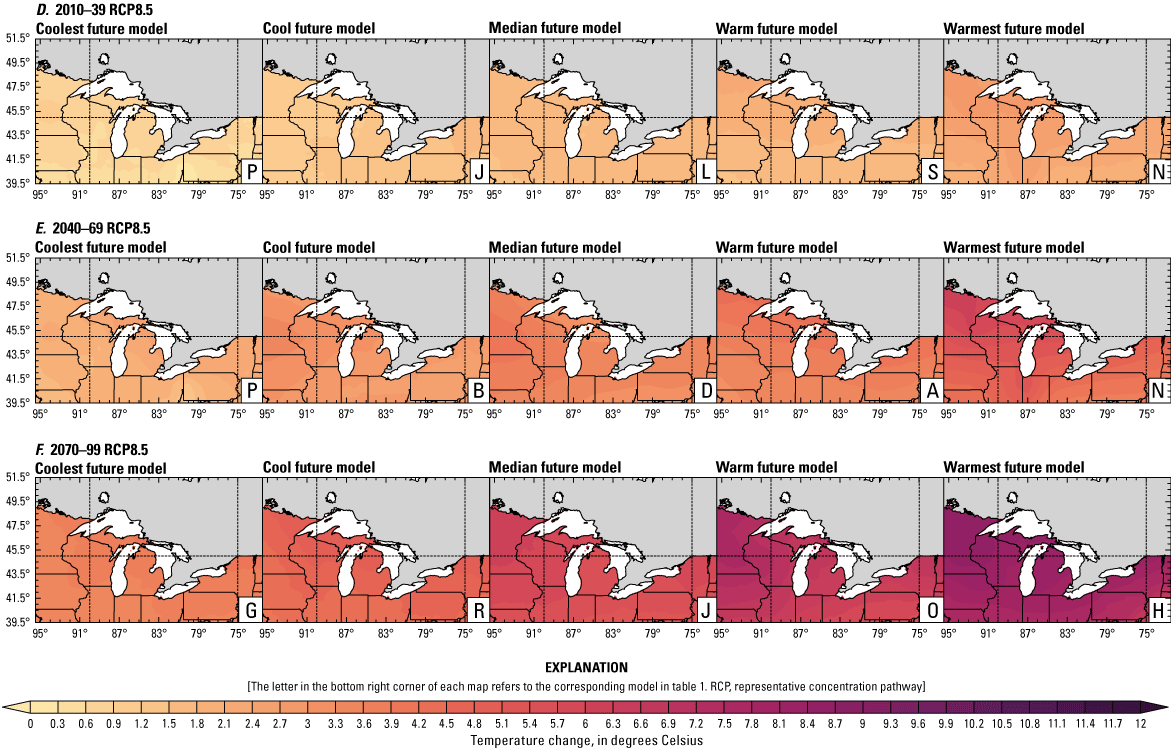
Annual mean 2-meter air temperature change (in degrees Celsius) in the downscaled climate simulations under emissions scenarios for representative concentration pathway 4.5 (RCP4.5) and representative concentration pathway 8.5 (RCP8.5) from the historical period (1971–2000) to the future periods. A, early 21st century (2010–39) for RCP4.5; B, mid-21st century (2040–69) for RCP4.5; C, late 21st century (2070–99) for RCP4.5; D, early 21st century (2010–39) for RCP8.5; E, mid-21st century (2040–69) for RCP8.5; F, late 21st century (2070–99) for RCP8.5.
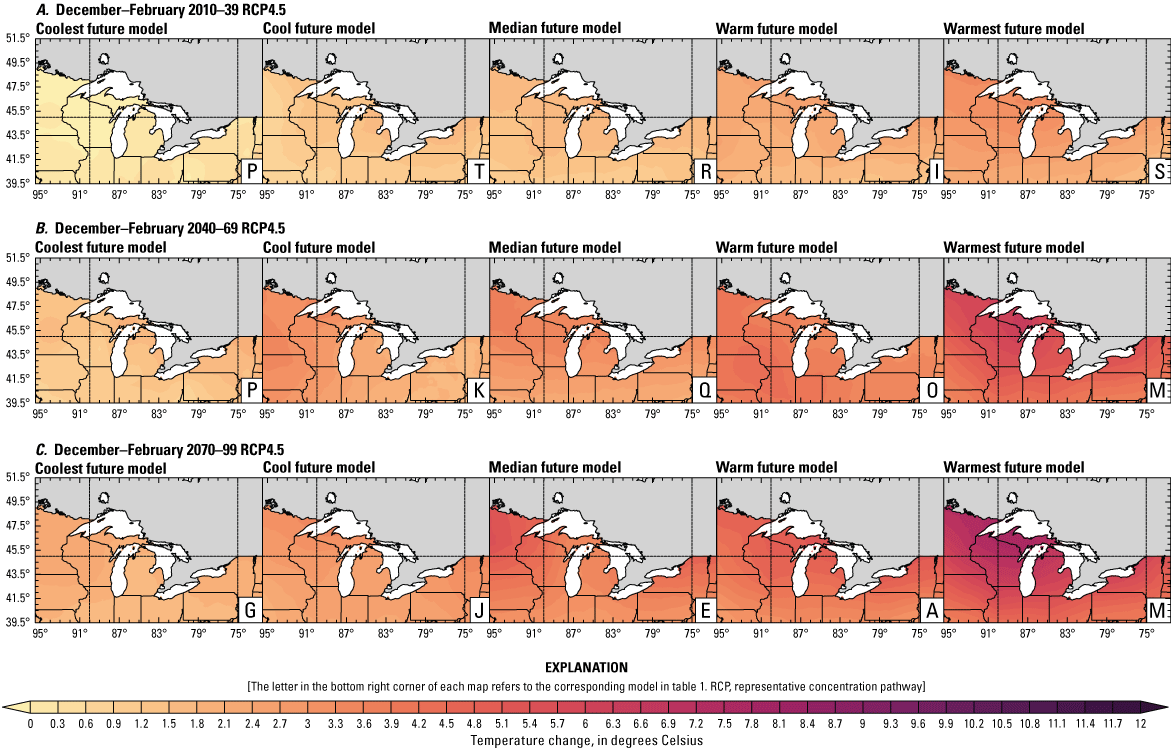
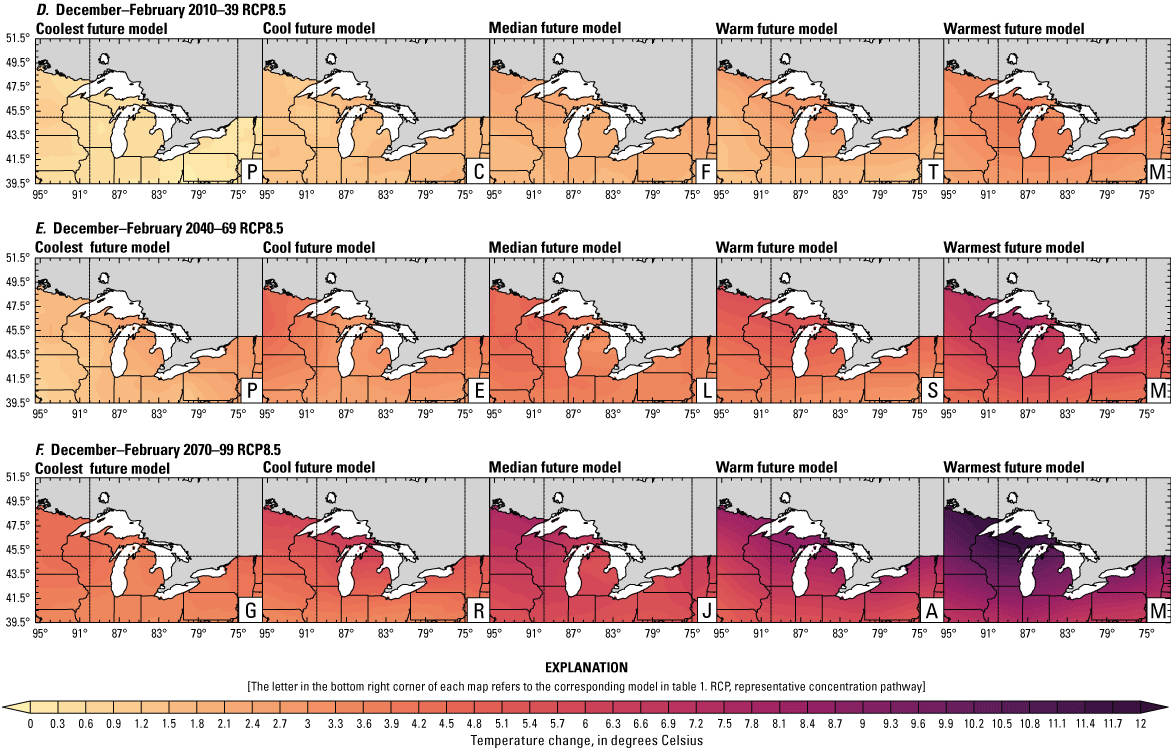
December–February mean 2-meter air temperature change (in degrees Celsius) in the downscaled climate simulations under emissions scenarios for representative concentration pathway 4.5 (RCP4.5) and representative concentration pathway 8.5 (RCP8.5) from the historical period (1971–2000) to the future periods. A, early 21st century (2010–39) for RCP4.5; B, mid-21st century (2040–69) for RCP4.5; C, late 21st century (2070–99) for RCP4.5; D, early 21st century (2010–39) for RCP8.5; E, mid-21st century (2040–69) for RCP8.5; F, late 21st century (2070–99) for RCP8.5.
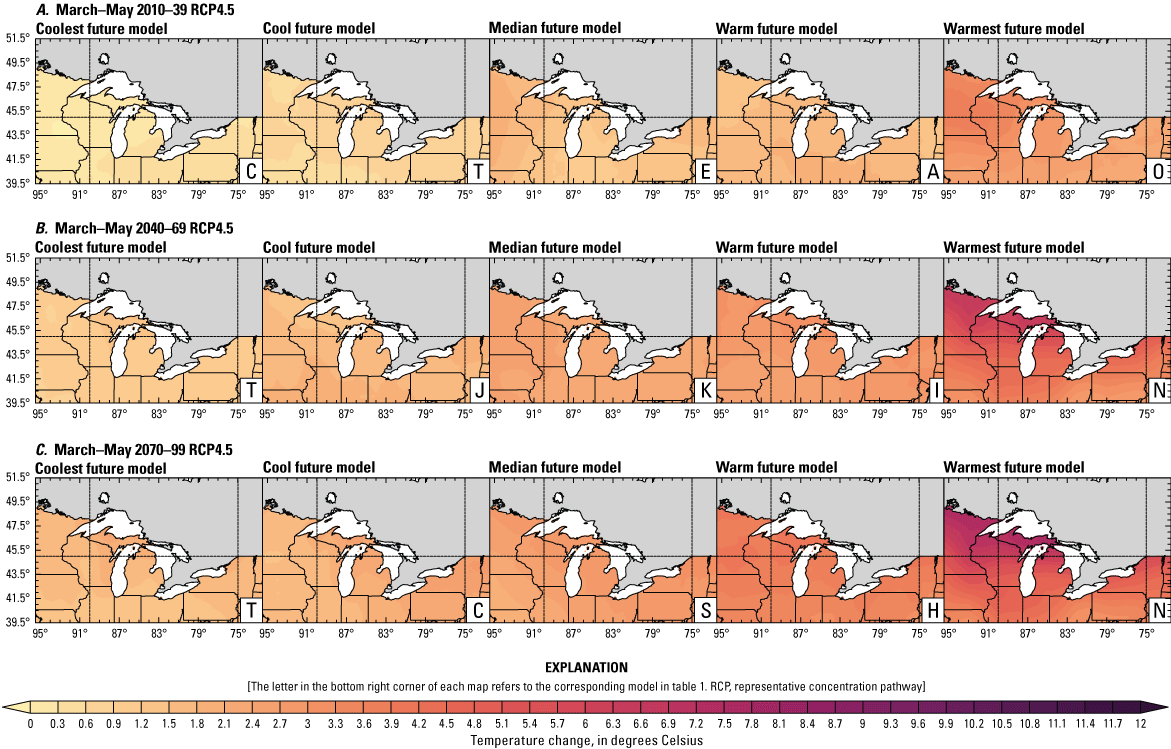
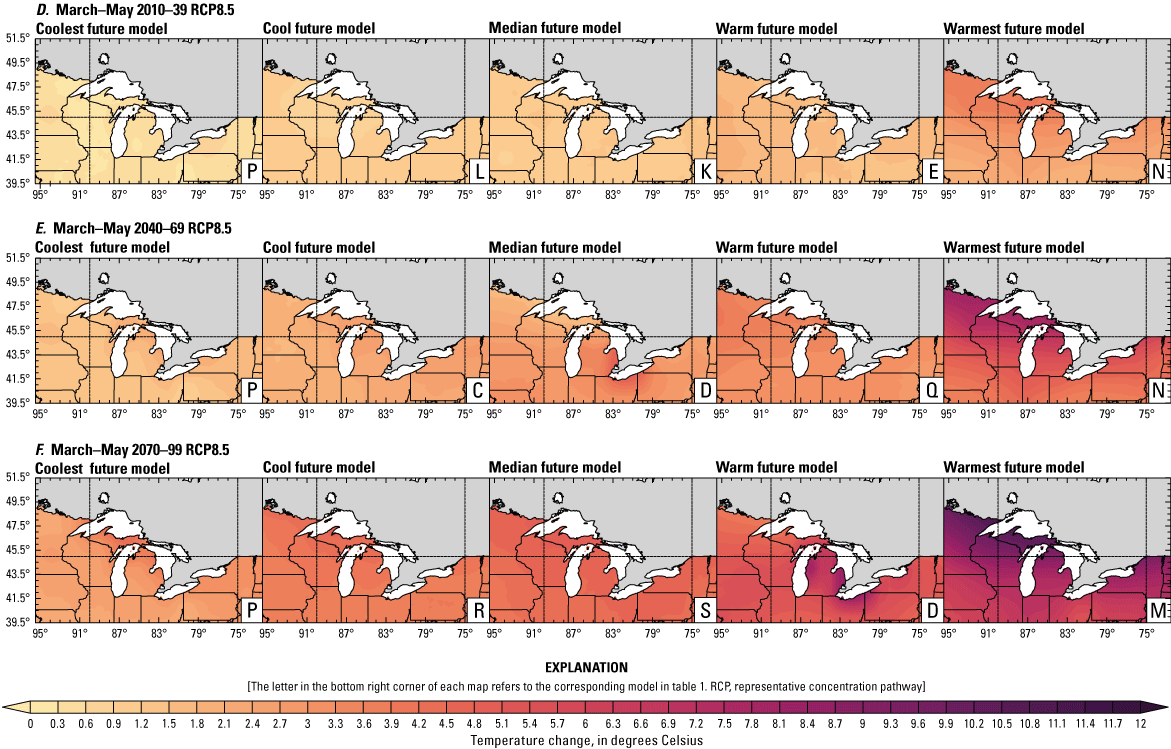
March–May mean 2-meter air temperature change (in degrees Celsius) in the downscaled climate simulations under emissions scenarios for representative concentration pathway 4.5 (RCP4.5) and representative concentration pathway 8.5 (RCP8.5) from the historical period (1971–2000) to the future periods. A, early 21st century (2010–39) for RCP4.5; B, mid-21st century (2040–69) for RCP4.5; C, late 21st century (2070–99) for RCP4.5; D, early 21st century (2010–39) for RCP8.5; E, mid-21st century (2040–69) for RCP8.5; F, late 21st century (2070–99) for RCP8.5.
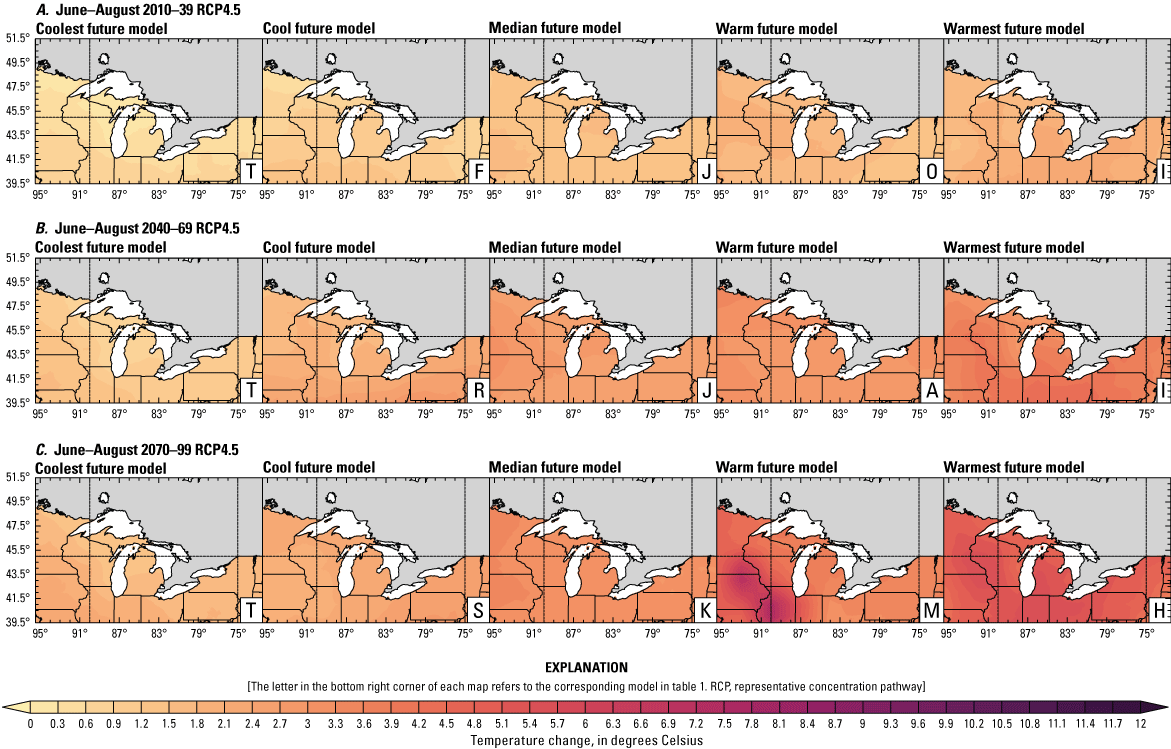
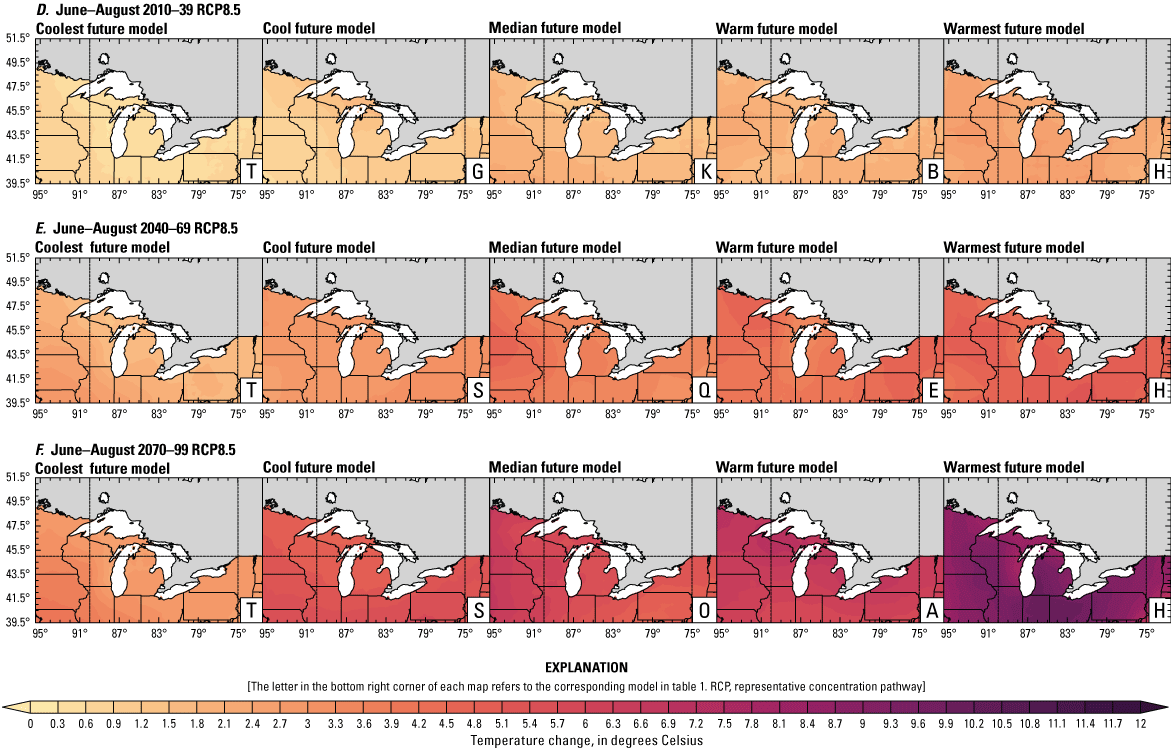
June–August mean 2-meter air temperature change (in degrees Celsius) in the downscaled climate simulations under emissions scenarios for representative concentration pathway 4.5 (RCP4.5) and representative concentration pathway 8.5 (RCP8.5) from the historical period (1971–2000) to the future periods. A, early 21st century (2010–39) for RCP4.5; B, mid-21st century (2040–69) for RCP4.5; C, late 21st century (2070–99) for RCP4.5; D, early 21st century (2010–39) for RCP8.5; E, mid-21st century (2040–69) for RCP8.5; F, late 21st century (2070–99) for RCP8.5.
Conversion Factors
For more information about this publication, contact:
Director, USGS Midwest Climate Adaptation Science Center
1954 Buford Avenue
St. Paul, MN 55108
For additional information, visit: https://www.usgs.gov/programs/climate-adaptation-science-centers/midwest-casc
Publishing support provided by the
Rolla Publishing Service Center
Suggested Citation
Embke, H.S., Nikiel, C.A., and Lyons, M.P., 2023, Potential effects of climate change on Acipenser fulvescens (lake sturgeon): U.S. Geological Survey Open-File Report 2021–1104–E, 41 p., https://doi.org/10.3133/ofr20211104E.
ISSN: 2331-1258 (online)
Study Area
Publication type | Report |
---|---|
Publication Subtype | USGS Numbered Series |
Title | Potential effects of climate change on Acipenser fulvescens (lake sturgeon) |
Series title | Open-File Report |
Series number | 2021-1104 |
Chapter | E |
DOI | 10.3133/ofr20211104E |
Year Published | 2023 |
Language | English |
Publisher | U.S. Geological Survey |
Publisher location | Reston, VA |
Contributing office(s) | Midwest Climate Adaptation Science Center |
Description | vi, 41 p. |
Country | United States |
Other Geospatial | Great Lakes |
Online Only (Y/N) | Y |
Additional Online Files (Y/N) | N |
Google Analytic Metrics | Metrics page |