Water Quality of Sand and Gravel Aquifers in McHenry County, Illinois, 2020 and Comparisons to Conditions in 2010
Links
- Document: Report (4.21 MB pdf) , HTML , XML
- Dataset: USGS National Water Information System database —USGS water data for the Nation
- Data Releases:
- USGS data release - Quality-assurance and quality-control data for discrete water-quality samples collected in McHenry County, Illinois, 2020
- USGS data release - Reconnaissance of Per- and Polyfluoroalkyl Substances (PFAS) in Selected Groundwater and Surface Water Sites in McHenry County, Illinois, 2020
- Download citation as: RIS | Dublin Core
Acknowledgments
The authors would like to thank Scott Kuykendall, of the McHenry County Department of Planning and Development, for his assistance with accessing each site and support of McHenry County groundwater resource monitoring. The authors also thank the Planning, Environment, and Development Committee and the McHenry County Board of McHenry County Planning and Development for their support of this study and the groundwater monitoring network. The authors also thank the McHenry County Conservation District for continuing to host the real-time groundwater monitoring sites on conservation property. Also, thanks to the Illinois State Geological Survey for allowing the U.S. Geological Survey (USGS) to use their existing monitoring wells to increase the distribution of groundwater monitoring sites. The USGS appreciates Daniel Abrams from the Illinois State Water Survey for contributing information regarding production wells throughout McHenry County. The USGS appreciates this opportunity to provide a water-quality update, along with continued support of the McHenry County Groundwater Monitoring Network.
The authors greatly appreciate the assistance from colleagues Monica Hall and Carolyn Soderstrom in sampling 47 monitoring wells and 3 surface-water sites during a pandemic and summertime heat. We also would like to thank our colleague Jennifer Sharpe for her assistance compiling figures for this report.
Abstract
McHenry County, Illinois, obtains most of its drinking water from shallow sand and gravel aquifers (groundwater). To evaluate this groundwater resource, the U.S. Geological Survey, in cooperation with McHenry County, Illinois, collected water-quality samples from 41 of 42 monitoring wells in the McHenry County Groundwater Monitoring Network and 4 monitoring wells from the U.S. Geological Survey National Water-Quality Assessment Project. Additionally, a subset of 12 monitoring wells was sampled and analyzed for pharmaceuticals and wastewater indicator compounds (WICs), collectively referred to as “contaminants of emerging concern” (CECs). Results from this 2020 study were compared to the 2010 results to assess changes in groundwater quality. Statistical analyses and chloride-bromide ratio analyses also were completed to assess changes in water quality.
Health-based benchmarks were exceeded for arsenic (about 24 percent; 11 of 45 monitoring wells), sodium (40 percent, 18 of 45), and manganese (about 2 percent, 1 of 45). Aesthetically based benchmarks were exceeded for dissolved solids (about 29 percent, 13 of 45), chloride (about 4 percent, 2 of 45), iron (about 87 percent, 39 of 45), and manganese (about 29 percent, 13 of 45). CECs were detected at low or estimated concentrations in 8 of the 12 (about 67 percent) monitoring wells analyzed.
In addition to sampling the groundwater monitoring wells, three surface-water-quality monitoring sites also were sampled and analyzed for pharmaceuticals and WICs to provide a preliminary assessment of the presence of CECs in the surface waters. CECs were detected in all three of the surface-water-quality monitoring samples collected, and WICs were more prevalent and more frequently detected than pharmaceutical compounds. These results provided a cursory understanding of the presence of CECs in surface waters and do not constitute a robust analysis of sources, seasonality, range of concentrations, persistence, or effects.
The 2020 groundwater-quality results had measurements of field properties, and concentrations of major ions, trace metals, and nutrients that were consistent with 2010 results with statistically significant increases for calcium, magnesium, and silica, and decreases for aluminum, ammonia, arsenic, barium, bromide, calcium, molybdenum, phosphate, specific conductance, sulfate, and dissolved solids. Increases generally were detected in the intermediate and deep parts of the sand and gravel aquifer, and decreases were detected in the shallow parts of the sand and gravel aquifer. The mixed distribution of increases and decreases among the various constituents and aquifer-depth groups could be reflecting dissolution and mobility of some of the redox sensitive constituents and dilution of some constituents in the shallow aquifer depths. These changes may be attributed to a combination of stable population of the past decade (2010–20), land-use management practices, and the recent wet years of 2017 through 2019 causing a dilution of the major ions in the shallow parts of the aquifer.
Introduction
McHenry County, Illinois, sources their drinking water primarily from the shallow (less than 300 feet [ft]) sand and gravel aquifers (groundwater). To evaluate this groundwater resource, a network of 42 groundwater monitoring wells (referred to as the “McHenry County Groundwater Monitoring Network” [MCGMN]) is used to monitor the water levels and water quality in the sand and gravel aquifers (fig. 1). In 2010, water-quality samples were obtained at 41 monitoring wells from the MCGMN, plus 5 additional monitoring wells available from the U.S. Geological Survey (USGS) National Water-Quality Assessment (NAWQA) Project were sampled and analyzed for field properties, major ions, trace metals, and nutrients. In 2010, samples from a subset of the monitoring wells also were analyzed for volatile organic compounds, pesticides, and herbicides. The results of that study are presented in Gahala (2017). In short, the findings of that report indicated health-based standards were exceeded for arsenic in 22 percent of the monitoring wells sampled, sodium in 37 percent of the monitoring wells sampled, and nitrates in 2 percent of the monitoring wells sampled. Aesthetically based standards were exceeded for total dissolved solids in 33 percent of the wells sampled, chloride in 4 percent of the wells sampled, iron in 85 percent of the wells sampled, and manganese in 30 percent of the wells sampled. Volatile organic compounds and pesticides were detected at trace levels in shallow wells (less than 46 ft below land surface) near urban settings. To identify changes in groundwater conditions in the shallow sand and gravel aquifer since 2010, the USGS, in cooperation with McHenry County, Illinois, and in collaboration with the McHenry County Planning and Development Committee, completed a decadal water-quality assessment of the sand and gravel aquifers in June–July of 2020.
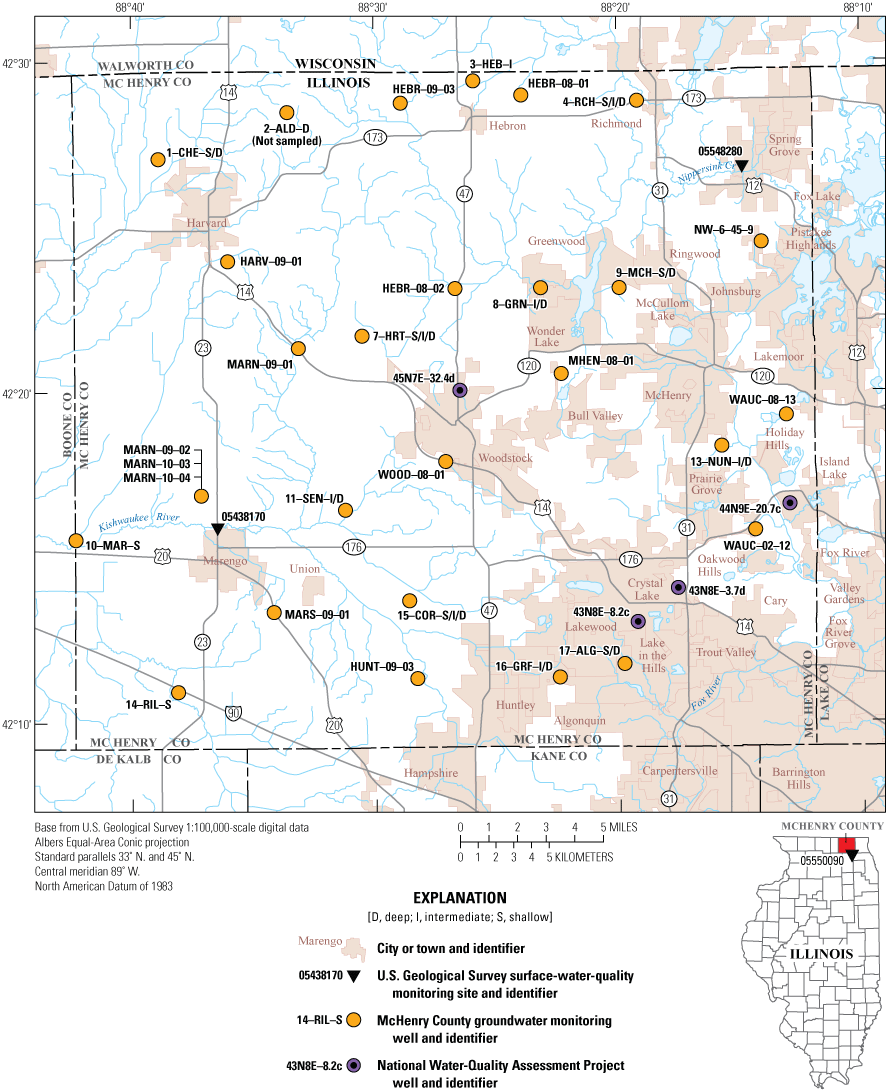
Groundwater monitoring wells of the McHenry County Groundwater Monitoring Network and U.S. Geological Survey National Water-Quality Assessment Project and three surface-water-quality monitoring sites sampled in June–July 2020 in McHenry County, Illinois.
The MCGMN monitoring wells, along with four USGS NAWQA Project shallow (less than about 46 ft) monitoring wells in urban areas, were sampled and analyzed for field properties, major ions, trace metals, and nutrients (table 1). Similar to the 2010 water-quality assessment, targeted monitoring wells (12 total) were sampled for contaminants of emerging concern (CECs). CECs can refer to many kinds of chemicals, including medicines, personal care products, cleaning products, and lawn care and agricultural products (U.S. Geological Survey, 2022). These CECs can enter our groundwaters and surface waters through runoff, septic systems, discharges from wastewater-treatment plants, and various industrial processes (U.S. Geological Survey, 2022). To identify if any CECs are present in the sand and gravel aquifers, pharmaceuticals and wastewater indicator compounds (WICs) were selected for analysis in the groundwater at targeted monitoring wells. In addition to the groundwater monitoring wells, three surface-water sites also were sampled and analyzed for CECs, and two of the sites included analyses for major ions, trace metals, and nutrients (table 1) to assess general stream-water quality downstream from wastewater-treatment plants and industries. Surface waters are the primary drainage ways for groundwater, stormwater runoff, and other land-use discharges. This preliminary assessment of the presence of CECs provides a cursory understanding of concentrations detected but does not constitute a robust analysis of sources, seasonality, range of concentrations, persistence, or effects in surface water.
Table 1.
Site information and water-quality sampling plan for field properties, major ions, trace metals, nutrients, and contaminants of emerging concern analyzed in the McHenry County Groundwater Monitoring Network, U.S. Geological Survey National Water-Quality Assessment monitoring wells, and three surface-water locations in Illinois, 2020.[Data collected for sites in this table can be accessed in the U.S. Geological Survey (USGS) National Water Information System database (U.S. Geological Survey, 2020b) using the USGS station identifiers. ft, foot; NAVD 88, North American Vertical Datum of 1988; X, analyzed; —, no detection; Co, County; CRN, Climate Response Network; IL, Illinois]
Aquifer unit names originated from Illinois State Water Survey (Meyer and others, 2013) and are used in this report for consistency purposes. See Gahala (2017) for further details on aquifer unit and monitoring well designations.
Purpose and Scope
The primary objectives of this study were to assess changes in the water quality as it pertains to human health and the environment and to identify potential effects of land use on water quality. This investigation provides an update on the water quality of the sand and gravel aquifers from the countywide network of groundwater monitoring wells and selected surface-water sites. The purpose of this report is to summarize and describe the findings from the analysis of water-quality samples collected during June and July of 2020 from the MCGMN, plus four NAWQA monitoring wells and three surface-water sites, and to complete the following:
-
• Describe the status of groundwater quality in 2020.
-
• Identify statistically significant differences in groundwater quality by applying the Wilcoxon signed-rank (WSR) test and paired Prentice-Wilcoxon (PPW) test.
-
• Provide an update on the sources of chloride (chloride-bromide analysis) and compare results to the 2010 data.
-
• Summarize the results of the water-quality analysis for CECs (pharmaceuticals and WICs) identified in samples from targeted groundwater monitoring sites and surface-water locations.
-
• Identify exceedances of drinking water standards and statistically significant differences in concentrations and potentially relate these results to land-use or other effects near the well.
Description of Study Area
McHenry County, Ill. (611 square miles), is 35 miles northwest of the Chicago metropolitan area. As described in Gahala (2017), the county is generally geographically split by type of land use. The western half of the county is dominated by agricultural land use, and the eastern half is primarily urban land use. Geologically, the land is described as hilly with moraines, kames, eskers, and outwash plains resulting from several glacial advances and retreats during the Quaternary Period (Hackett and McComas, 1969; Berg and others, 1997; Stiff and Hansel, 2004). McHenry County is divided by two hydrologic drainage basins, the Fox River drainage basin, which drains towards the east, and the Kishwaukee River drainage basin, which drains towards the west (Nicholas and Krohelski, 1984; Berg and others, 1997; Illinois State Water Survey, 2022; Gahala, 2017, fig. 3).
Precipitation ranged from 27.07 inches (in.) in 2012 to 49.9 in. in 2018, with an average of 44.51 in. over the decade (2010–20) according to the Global Historical Climate Network observation in Woodstock, Ill. (Woodstock 5NW) (National Centers for Environmental Information, 2022a). The legacy climatological period from 1981 to 2010 from weather observation station Woodstock 5NW, in McHenry County, Ill., indicates an average annual precipitation of 36.62 in. (National Centers for Environmental Information, 2022b). The most recent standard climatological period (1991–2020) indicates an average annual precipitation of 38.68 in. (National Centers for Environmental Information, 2022c), and the latest 15-year period from 2006 to 2020 indicates an average annual precipitation of 41.18 in. (National Centers for Environmental Information, 2022d). The average annual precipitation, calculated from the daily values from 2010 to 2020 from data downloaded for the observation station Woodstock 5NW is slightly greater than the 2006–20 average annual precipitation (44.51 in.) for the decade. Increases in precipitation can affect water quality by increasing the amount of runoff and infiltration of various land-use inputs through mobilization of constituents into nearby surface waters (Hatfield and Prueger, 2004) and potentially imparting geochemical changes and transport within an aquifer.
Aquifers used to supply drinking water in McHenry County were mapped and described in detail by Meyer and others (2013). Drinking water supplies for McHenry County are pumped from deeper bedrock aquifers such as the Cambrian-Ordovician, Silurian dolomite, and the shallow sand and gravel aquifers within glacial drift deposits (Woller and Sanderson, 1976). Directions of groundwater flow were determined by Meyer and others (2013) and Gahala (2017) and are briefly summarized in this section. Groundwater is replenished (recharge) from precipitation that falls onto the land surface and infiltrates through the soil and sediment to the water table, where it flows downward and laterally through hydraulically connected sediments over time. Some of the groundwater flows laterally and discharges into local surface-water bodies. Deeper parts of the sand and gravel aquifers that are overlain by a low-permeability clay (till) can receive recharge through downward transport through saturated clays or through preferential, permeable flow paths made by thin or discontinuous clay deposits.
This study focuses on the water quality within the shallow sand and gravel aquifers. The aquifer units within the shallow sand and gravel aquifers that the monitoring wells represent are described in detail in Meyer and others (2013) and Gahala (2017) (table 1). Two wells are screened in the upper 10 ft of the Shallow Bedrock Aquifer. The source of the water is recharged from the sand and gravel aquifers.
The MCGMN and NAWQA wells are used to monitor for the effects of various land uses and changes. Samples collected from the MCGMN and NAWQA wells give a snapshot of water quality at shallow, intermediate, and deep depths. The depths of the MCGMN and NAQWA monitoring wells range from 20.3 to 344.4 ft and are generally comparable to the depth range of production and private (residential) wells extracting drinking water from the shallow sand and gravel aquifers. According to the Illinois State Water Survey, estimates from the ILWATER database indicate about 58 percent of production wells are extracting groundwater from the sand and gravel aquifers at depths of 16 to 272 ft. About 69 percent of private wells are extracting groundwater from sand and gravel aquifers at depths from 8 to 372 ft (Daniel Abrams, Illinois State Water Survey, written commun., March 23, 2022). It is important to note that these estimates from the ILWATER database are uncertain because the database does not account for wells that have not been abandoned (sealed) or categorized as both bedrock and unconsolidated (Daniel Abrams, Illinois State Water Survey, written commun., March 23, 2022).
The 2020 population estimate for McHenry County was 310,229, compared to the 2010 population of 308,760 (U.S. Census Bureau, 2021). The USGS has estimated water use for the United States every 5 years since 1950 at the State level, and since 1985 at the county level. The most recent estimate of water use was for 2015 (U.S. Geological Survey, 2020a). The water-use compilation includes data from production wells, domestic-supply wells, irrigation wells, and other sources (industrial, mining, livestock, and aquaculture) and is reported at the county level. The population served and water-use estimates for 2015 indicate that total groundwater withdrawals have decreased by about 9 percent from 2010 (U.S. Geological Survey, 2020a; table 2). The USGS is transitioning from previous approaches of 5-year annual compilations to a nationally consistent modeling platform to improve temporal and spatial discretization of water use that is planned to allow forecasting capability. Water-use data were not available for 2020 at the time of this publication.
Table 2.
Water use by category, volume of groundwater withdrawals, and population served, McHenry County, Illinois, 2010 and 2015.[Data from U.S. Geological Survey, 2020a. Mgal/d, million gallons per day; —, no data or not applicable]
Includes industrial, mining, livestock, and aquaculture. Descriptions of estimation methodology are available at https://water.usgs.gov/watuse/data/index.html.
As of 2009, land use in the county was 61 percent agriculture, 11 percent open spaces, and 16 percent residential, and the remaining land use consisted of government/institutional, commercial retail, industrial, earth extraction, and some designated as “vacant” (McHenry County Planning and Development, 2020). It is estimated that by 2030, agricultural land use will be reduced to 45 percent, and residential, open spaces, and spaces designated as “environmentally sensitive” together will account for 51 percent of the land use (McHenry County Planning and Development, 2020).
Previous Water-Quality Investigations
Countywide water quality of the sand and gravel aquifers of McHenry County was assessed from sampling of municipal supply wells in 1976 (Woller and Sanderson, 1976) and nearly quarterly sampling of residential wells from April 1979 through April 1980 (Nicholas and Krohelski, 1984), which included major ions and nutrients. Results from these studies indicated concentrations of major ions and nutrients were not substantially variable and were generally consistent across seasons. Dissolved iron had the greatest amount of fluctuation but could be affected by other factors, such as age of the residential well and piping. Health and aesthetically based water-quality benchmarks were exceeded for sodium, nitrates, iron, manganese, and dissolved solids in residential wells sampled (Nicholas and Krohelski, 1984). In 2010, 41 of 42 monitoring wells in the MCGMN plus 5 NAWQA monitoring wells were sampled for field properties, major ions, metals, trace elements, and nutrients (Gahala, 2017). Health and aesthetically based water-quality benchmarks were exceeded for arsenic, sodium, nitrate (as nitrate plus nitrite), manganese, dissolved solids, chloride, and iron.
Methods of Study
This section describes methods used to collect and analyze discrete water-quality samples, including sampling procedures, data analysis, and quality assurance and quality control (QAQC). The statistical analysis applied to the water-quality results also is described.
Water-Quality Sampling
The MCGMN consists of 42 monitoring wells, of which 37 are equipped with pressure transducers that continuously monitor the groundwater levels (1 reading every 15 minutes). These data are transmitted using satellite telemetry to the USGS National Water Information System database (U.S. Geological Survey, 2020b) Water-quality samples were collected from 41 of the 42 monitoring wells in the MCGMN and 4 NAWQA monitoring wells during June–July 2020 (fig. 1, table 1). The NAWQA monitoring wells were included to assess effects from urbanization. Two monitoring wells (2–ALD–D from MCGMN and 45N9E–7.6a from NAWQA) were planned to be sampled in 2020 but were not sampled because of site conditions and well integrity issues. All groundwater monitoring wells were sampled for field properties, major ions, trace metals, and nutrients. Field properties included dissolved oxygen, pH, dissolved solids, specific conductance, water temperature, turbidity, and alkalinity. Additionally, 12 monitoring wells were sampled for the analysis of CECs. Water-quality results can be retrieved from the USGS National Water Information System database using the station identifiers in table 1. The chloride-bromide ratio analysis from Gahala (2017) was used to help select which wells would be sampled for CECs. Based on the chloride-bromide ratio analyses, 9 of the 12 wells were identified as potentially affected by sewage, and the remaining 3 wells were considered control wells and were not identified as being affected by sewage (Gahala, 2017). Water-quality sampling procedures generally adhered to the USGS “National Field Manual for the Collection of Water-Quality Data” (U.S. Geological Survey, variously dated).
Surface-water samples also were collected in 2020 to preliminarily assess the presence of CECs downstream from wastewater-treatment plants and other industrial discharges. Five grab samples were collected along the cross-sectional transect of each stream and composited with a precleaned and prerinsed churn. This approach slightly deviated from the recommended method described in the National Field Manual (U.S. Geological Survey, variously dated), where streams greater than 5 ft in width should have a minimum of 10 sample points. Cross-sectional field properties were measured within the stream with a multiparameter device at intervals of 2–5 ft at each surface-water-quality monitoring location to determine if the stream was well mixed at that location. Water-quality properties (major ions, trace elements, and nutrients) were added at two of the three surface-water locations to assess general water quality in the two streams that contribute to downstream (beyond McHenry County) drinking water sources.
Water-quality samples were collected following the methods described in the USGS “National Field Manual for the Collection of Water-Quality Data” (U.S. Geological Survey, variously dated), and field alkalinities were completed in the field using the inflection-point method. Major ions, trace metals, and nutrients were analyzed by the methods described in Fishman (1993), Fishman and Friedman (1989), and Garbarino and others (2006). WICs are a general category for anthropogenically derived chemicals that include some pharmaceuticals, pesticides, and some volatile organic compounds all quantified in parts per billion (micrograms per liter). Pharmaceuticals are quantified in parts per trillion (nanograms per liter). Pharmaceuticals and WICs were analyzed by the methods described in Furlong and others (2008 and 2014) and Zaugg and others (2006). Water-quality samples were shipped with appropriate sample preservation to the USGS National Water Quality Laboratory (NWQL) in Denver, Colorado, for analysis. The NWQL uses method reporting conventions for establishing the minimum concentration greater than which a quantitative measurement can be made (Oblinger Childress and others, 1999). These reporting conventions are the method reporting level and the laboratory reporting level. The method reporting level is defined by the NWQL as the smallest measured concentration of a substance that can be reliably measured using a given analytical method. The method detection level is the minimum concentration of a substance that can be measured and reported with 99-percent confidence that the concentration is greater than zero (Oblinger Childress and others, 1999; Barr and Davis, 2010). The laboratory reporting level is the interim reporting level, which is typically two times the method detection level to minimize false negative risks and is the smallest concentration of a chemical that can be reported by the laboratory (Williams and others, 2015). QAQC results are summarized in appendix 1 and are groundwater focused. No QAQC samples were collected for the preliminary CEC assessment in the surface water. QAQC samples include equipment blanks, field blanks, and replicates (replicate of the primary environmental sample) and were collected almost weekly throughout the duration of the sampling effort. For a total of 12 QAQC samples, 2 equipment blanks, 5 field blanks, and 5 replicates were collected. The full results of the QAQC samples and the full list of CECs sampled for are summarized in Gahala and Gruhn (2022).
The water-quality results of the 2020 data are compared to health-based and aesthetically based benchmarks in water-quality samples established by the U.S. Environmental Protection Agency (EPA), such as the drinking water advisories (DWAs) and established maximum contaminant level (MCL) and the secondary maximum contaminant level (SMCL) (U.S. Environmental Protection Agency, 2009a, b, 2018). Aesthetically based benchmarks are derived from EPA SMCLs, which are nonregulated, recommended criteria that establish taste and odor quality. Surface-water-quality results also were compared to aquatic ambient water-quality criteria established by the EPA to assess suitability for aquatic life (U.S. Environmental Protection Agency, 2021b).
Statistical Analysis
To identify changes in water quality between 2010 and 2020, two analyses were completed. Using paired samples, differences between 2010 and 2020 were assessed across all wells for each constituent. For constituents that did indicate a statistically significant difference across all wells, differences between 2010 and 2020 also were assessed for three groups of wells in either deep, intermediate, or shallow aquifers. A total of 20 constituents were measured in 43 wells in 2010 and 2020. The wells also were grouped according to aquifer depth; 10, 19, and 14 wells were designated as “shallow” (13.6–58.7 ft below ground surface), “intermediate” (62.3–120.6 ft below ground surface), and “deep” (139–344 ft below ground surface), respectively. Of the constituents, nine had at least one censored (less than the reporting level) observation. An alpha level of 0.05 was used for determining statistical significance. All statistical analyses were completed using the R statistical software program (R Core Team, 2019), specifically the NADA (Lopaka and Helsel, 2020). NADA2 (Julian and Helsel, 2021) and Wilcox (R Core Team, 2021) R packages, which include statistical tests for censored data.
The applicable statistical test depended on the presence of censored values in the dataset. The WSR test (Helsel, 2012) was used to assess changes when the data were all uncensored values. The PPW test was used when data were a mix of censored and uncensored values. Both tests are nonparametric, require no assumptions about the distribution of the data, and have a null hypothesis that the difference between paired samples is zero. Rejection of the null hypothesis indicates samples collected in 2020 differ from those collected in 2010. The PPW test is a score test and allows for left-censored values to have multiple reporting levels, meaning recensoring data to the highest reporting level is not required (Helsel, 2012). These two tests also were used when testing for differences in concentration between 2010 and 2020 for each aquifer group. Because three comparisons (one for each aquifer group) were made for each parameter, probability values (p-values) from the WSR and PPW tests were adjusted using the Benjamini and Hochberg correction, which controls the false discovery rate when completing multiple comparison tests (Benjamini and Hochberg, 1995). The combination of testing for differences between 2010 and 2020 across all wells and testing for differences within each aquifer group helped to identify at what depth changes were likely.
2020 Water Quality
Results of the 2020 decadal water-quality sampling are presented in this section and include field properties, major ions, trace metals, and nutrients. Selected monitoring wells were sampled for CECs, which include pharmaceuticals, pesticides, and WICs. The results for three surface-water sites sampled also are included in tables in this report. The surface-water results did not have any detected concentrations of major ions, metals, or nutrients greater than any aquatic ambient water-quality criteria. Groundwater water-quality results, including the implications on the suitability for drinking water, are discussed in the following sections. The following sections compare constituents with the health-based standards and aesthetically based recommendations by the EPA and include the enforceable MCLs, nonenforceable SMCLs, and DWA (U.S. Environmental Protection Agency, 2009a, 2018; table 3).
Table 3.
U.S. Environmental Protection Agency water-quality thresholds, number of wells exceeding water-quality thresholds in samples collected in 2020, and statistical description (maximum, minimum, median).[MCL, maximum contaminant level; µg/L, microgram per liter; DWA, drinking water advisory; mg/L, milligram per liter; SMCL, secondary maximum contaminant level; >, greater than]
Water-quality standards and benchmark concentrations are from U.S. Environmental Protection Agency (2018).
Data summarized from U.S. Geological Survey (2020b).
Field Properties
Results for field properties are presented in table 4. Dissolved oxygen concentrations at groundwater wells ranged from 0.0 to 7.0 milligrams per liter (mg/L) with a median 0.20 mg/L but were typically (80 percent) less than 1.0 mg/L. According to McCallum and others (2008), denitrification becomes more favorable at a dissolved oxygen concentration of less than 2.0 mg/L. Dissolved oxygen at surface-water sites ranged from 8.4 to 14.3 mg/L.
Table 4.
Results for field properties for the McHenry County Groundwater Monitoring Network wells, 4 National Water-Quality Assessment Project monitoring wells, and 3 surface-water sites, McHenry County, Illinois, 2020.[Data available from U.S. Geological Survey, 2020b. mg/L, milligram per liter; std, standard; µS/cm, microsiemens per centimeter at 25 degrees Celsius; °C, degree Celsius; NTU, nephelometric turbidity unit; CaCO3, calcium carbonate; IL, Illinois; —, no data]
The pH collected at groundwater wells ranged from 6.5 to 7.6 standard pH units with a median of 7.2, and field alkalinities ranged from 268 to 429 mg/L as calcium carbonate with a median of 340 mg/L as calcium carbonate. Surface-water sites had higher pH values than wells and ranged from 8.0 to 8.9; no field alkalinities were measured at the surface-water sites.
Specific conductance measured at groundwater sites ranged from 489 to 2,343 microsiemens per centimeter at 25 degrees Celsius (µS/cm) with a median of 719 µS/cm, and specific conductance at surface-water sites ranged from 688 to 803 µS/cm. Some of the groundwater sites had elevated measurements of specific conductance and may be affected by the application of salt and salt brines on roads that can infiltrate into the groundwater. Elevated specific conductance was especially noticeable at shallow well sites that are closer to roadways.
Water temperature in groundwater wells ranged from 11.8 to 25.3 degrees Celsius (°C) with a median of 12.85 °C. Most wells were in the 11–16 °C range, but two wells were considerably higher and more similar to surface-water sites (17–ALG–S and 4–RCH–S), potentially from heating of the pump discharge line and flow-through chamber because of incomplete shading on sunny days or from the lower pumping rates allowing the water to warm within the discharge lines. Surface-water sites had water temperatures ranging from 21.3 to 28.2 °C, which is typical of many Midwest streams and rivers in June and July.
Turbidity at groundwater sites was generally low and ranged from 0.4 to 77.0 nephelometric turbidity units (NTU), and the median was 3.4 NTU. No turbidity measurements were made at surface-water sites.
Major Ions
Major ions were analyzed in the groundwater samples and two surface-water samples to compare to applicable drinking water standards, determine changes in groundwater sources, and identify potential land-use effects. Constituents included calcium, magnesium, potassium, chloride, sodium, dissolved solids, bromide, fluoride, silica, and sulfate. Results from the analysis of these samples are presented in table 5 and further described in the following paragraphs.
Table 5.
Results for concentrations of major ions from samples collected from the McHenry County Groundwater Monitoring Network wells, 4 National Water-Quality Assessment wells, and 3 surface-water sites in McHenry County, Illinois, 2020.[Data available from U.S. Geological Survey, 2020b. mg/L, milligram per liter; <, less than reporting level; E, estimated; —, no data; IL, Illinois]
Calcium in monitoring wells ranged from 41.8 to 184 mg/L with a median of 85.9 mg/L, and at the two surface-water sites, results were 44.5 mg/L and 72.5 mg/L. Magnesium in wells ranged from 27.8 to 95.0 mg/L with a median of 44.9 mg/L, and concentrations in surface-water sites were 34.8 mg/L and 37.9 mg/L. Calcium and magnesium are dissolved from soils, sediments, and rock, particularly limestone and dolomite; these constituents can cause water hardness and scale formation on pipes and plumbing (National Ground Water Association, 2010).
Potassium in groundwater wells ranged from 0.83 to 8.00 mg/L with a median of 1.54 mg/L, and concentrations in surface-water sites were 2.39 mg/L and 2.73 mg/L. Potassium is not only commonly detected in clays, rocks, and soils, but also can be detected in fertilizers (Mullaney and others, 2009). High concentrations of potassium (greater than 500 mg/L) are usually indicative of a road salt source, especially if potassium chloride is applied as a deicer (National Ground Water Association, 2010; Gahala, 2017).
Sodium ranged from 4.3 to 180 mg/L with a median of 14.64 mg/L, and concentrations in surface-water sites were 29.3 mg/L and 52.3 mg/L. High occurrences of sodium were often incidental with high occurrences of chloride, which could indicate that sodium chloride is being used as a deicer for roadways.
Bromide in the groundwater wells ranged from an estimated 0.011 to 0.254 mg/L with a median of 0.031 mg/L, and concentrations at the two surface-water sites were 0.032 mg/L and 0.036 mg/L. Fluoride in groundwater ranged from 0.05 to 0.68 mg/L with a median of 0.29 mg/L, and concentrations at the surface-water sites were 0.14 mg/L and 0.16 mg/L. Silica in groundwater ranged from 8.8 to 25.3 mg/L with a median of 19.96 mg/L, and concentrations at the surface-water sites were 1.1 mg/L and 15.9 mg/L. Bromide is a trace element in seawater, or naturally dissolved from sedimentary rocks such as evaporites, carbonates, and shales (Salameh and others, 2016). Anthropogenic sources of bromide can come from septic tanks, deicers, agricultural chemicals, solvents, and gasoline additives (Davis and others, 1998). Fluoride in groundwater is from weathering and leaching of fluoride-bearing minerals from rocks and sediments (Jha and others, 2013). Anthropogenic sources of fluoride can include coal burning, oil refining, steel productions, brick-making industries, and phosphatic fertilizer plants (Jha and others, 2011).
Sulfate from wells ranged from 0.03 to 97.7 mg/L with a median of 29.7 mg/L, and concentrations at the two surface-water sites were 24.5 mg/L and 30.7 mg/L. Hydrogen sulfides commonly are associated with a “rotten egg” odor and can impart a foul smell at higher concentrations (Agency for Toxic Substances and Disease Registry, 2011). Sniff tests at the time of sampling can often determine if there is a presence of hydrogen sulfides. Additional discussion later in this report relates these values to health-based benchmarks, implications to drinking water suitability, and aquatic life criteria for surface water.
Results for major ions did not exceed any health-based benchmarks except sodium, which exceeded the DWA of 20 mg/L in 40 percent (18 of 45 groundwater sites) of samples (fig. 2, table 5). Sources of sodium include dissolution of sediments and rocks, road salt, and septic-system leachate solutions (U.S. Environmental Protection Agency, 2002; Panno and others, 2006). Excess ingestion of sodium can increase blood pressure in at-risk individuals (Centers for Disease Control and Prevention, 2022). DWAs are not legally enforceable, and the 20 mg/L benchmark applies only to people on a 500-milligram-per-day restricted sodium diet (U.S. Environmental Protection Agency, 2018).
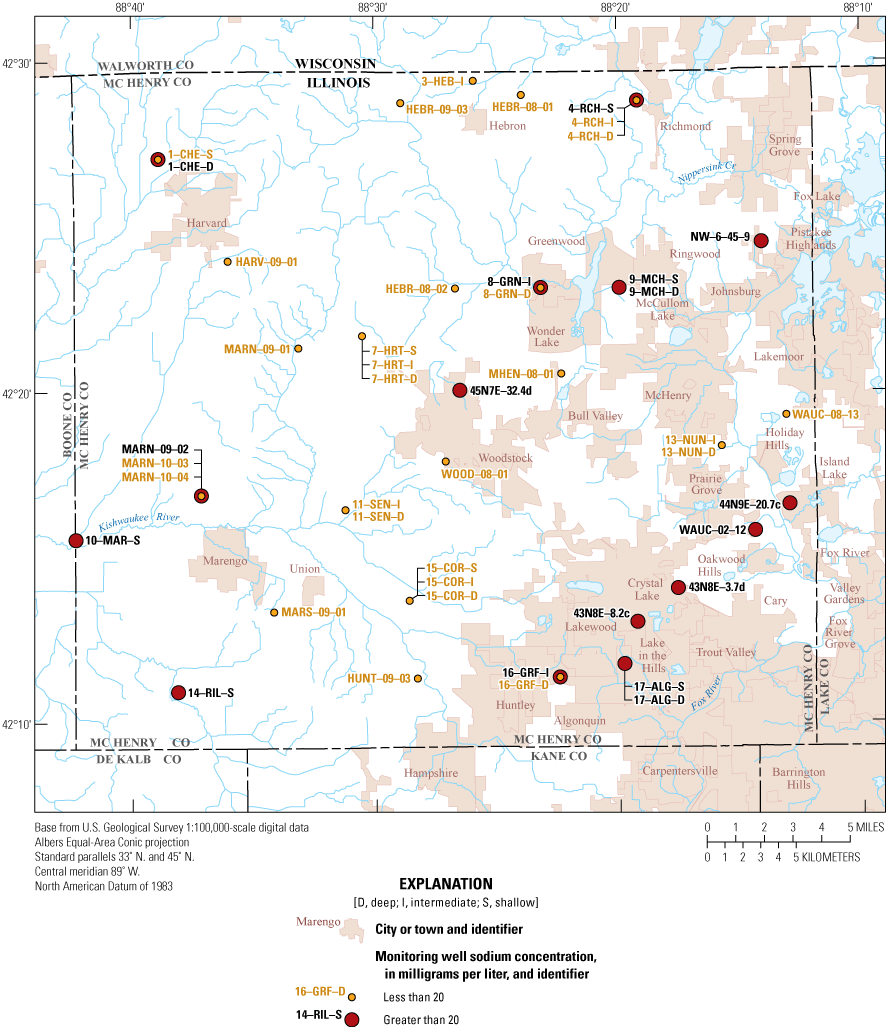
Distribution of sodium concentrations in samples from the McHenry County Groundwater Monitoring Network wells and the National Water-Quality Assessment Project monitoring wells, McHenry County, Illinois, 2020.
Dissolved solids are a measure of the dissolved ion concentrations in water. Groundwater samples ranged from 272 to 1,358 mg/L with a median of 417 mg/L, and concentrations at surface-water sites were 385 and 403 mg/L. The EPA has established an SMCL for dissolved solids because they can aesthetically affect groundwater, leave objectionable tastes, and have laxative effects when consumed at concentrations greater than 1,000 mg/L (table 3). Additionally, higher concentrations of dissolved solids can cause foaming and corrosion of metals. Dissolved solids can distinguish types of aquifers or indicate contamination from landfill leachate or road salt (Clark and Piskin, 1977). Shallower wells generally having higher dissolved solids concentrations than deeper wells (fig. 3); 29 percent of the groundwater wells (13 of 45 sites) exceeded the SMCL of 500 mg/L and 4 percent (2 of 45 sites) exceeded the 1,000-mg/L concentration (fig. 4). The two surface-water sites had similar concentrations of dissolved solids and did not exceed any thresholds of concern.
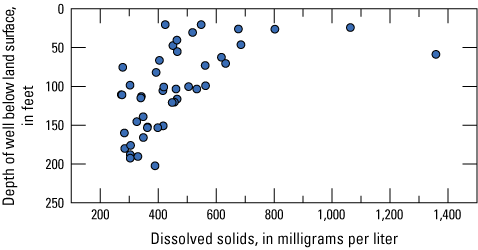
Dissolved solids concentrations by well depth in the McHenry County Groundwater Monitoring Network wells and the National Water-Quality Assessment Project monitoring wells, McHenry County, Illinois 2020.
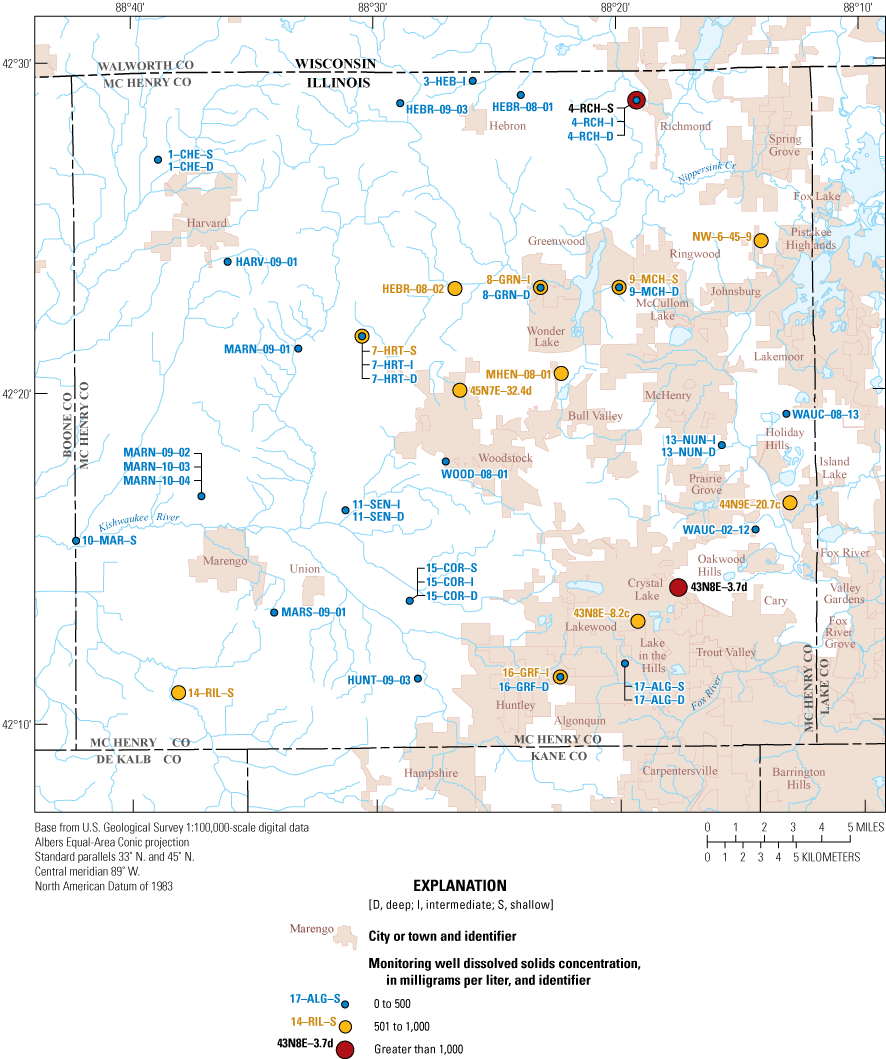
Distribution of dissolved solids concentrations in the McHenry County Groundwater Monitoring Network wells and the National Water-Quality Assessment Project monitoring wells, McHenry County, Illinois, 2020.
Chloride in groundwater ranged from 0.55 to 517 mg/L with a median of 16.9 mg/L, and concentrations at surface-water sites were 52.8 mg/L and 89.6 mg/L (table 5). Chloride was variable across wells, and the highest concentrations were near roadways. High concentrations of chloride are often attributed to road salt being applied as a deicer during winter weather events. Chloride has an SMCL of 250 mg/L, and greater concentrations can impart a salty taste on drinking water and can contribute to the corrosion of infrastructure (Shi and others, 2009; Pieper and others, 2018). Detections of chloride were variable across the MCGMN, and the highest concentrations were detected near roadways. A total of 4 percent of the wells (2 of 45 wells) exceeded the SMCL, and several shallow wells have elevated concentrations of chloride (fig. 5).
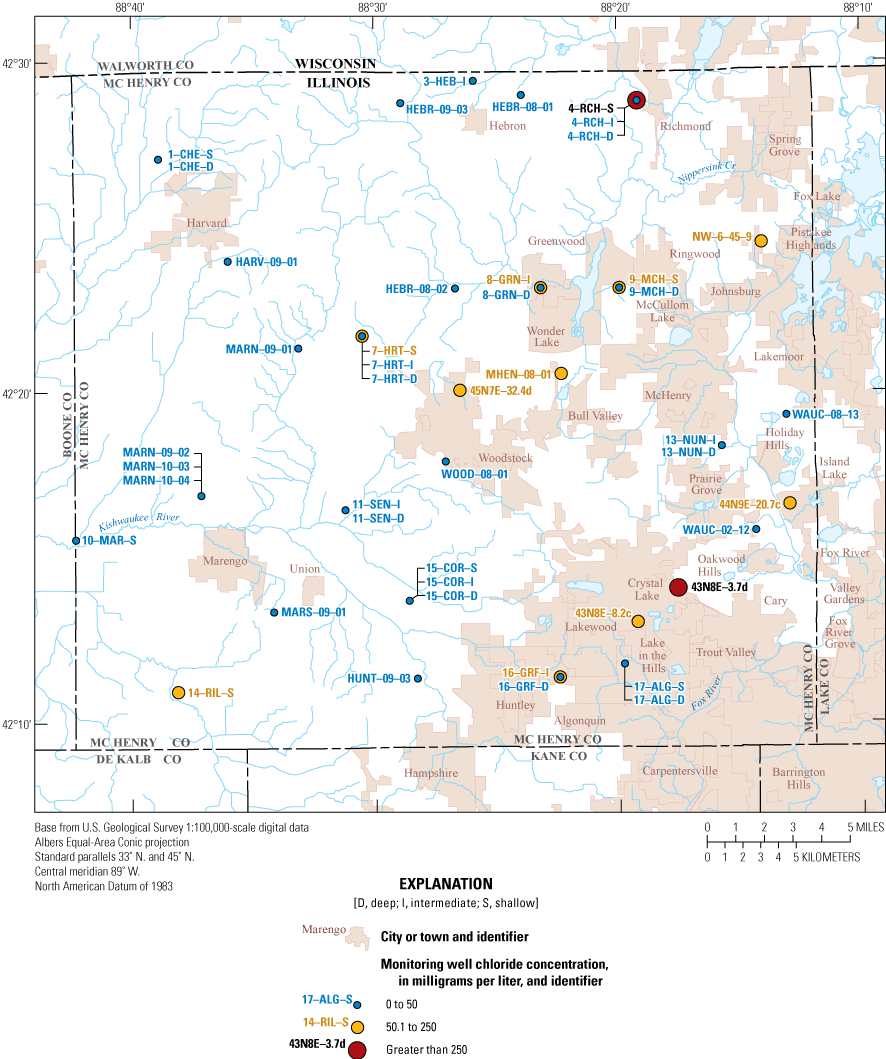
Distribution of chloride concentrations in the McHenry County Groundwater Monitoring Network wells and the National Water-Quality Assessment Project monitoring wells, McHenry County, Illinois, 2020.
Trace Metals
Metals and trace elements are naturally occurring in the environment, although natural concentrations can vary depending on local geology and contributions from anthropogenic activities (U.S. Geological Survey, 2019). Some metals are essential nutrients, but all metals can be toxic at some level, and for some metals, it only takes small amounts to cause toxicity (U.S. Environmental Protection Agency, 2022a). During the 2020 sampling campaign, a variety of metals and trace elements were analyzed for, and these results are presented in table 6. These results are discussed in relation to health-based benchmarks and compared to the 2010 results later in this report to determine suitability of the groundwater and surface water for drinking and the potential implications on aquatic life in surface water. In general, most metals and trace elements were less than the reporting level or detected at low concentrations and did not exceed any health-based benchmarks (table 3); however, arsenic, iron, and manganese did exceed a benchmark and are discussed individually in this section.
Table 6.
Results for concentrations of trace metals from samples collected from the McHenry County Groundwater Monitoring Network wells, 4 National Water-Quality Assessment wells, and 2 surface-water sites in McHenry County, Illinois, 2020.[Data available from U.S. Geological Survey, 2020b. µg/L, microgram per liter; <, less than reporting level; E, estimated; IL, Illinois; —, no data]
Results for arsenic in groundwater and surface water were variable and ranged from less than 0.20 to 70.3 micrograms per liter (µg/L). Arsenic is a naturally occurring element that is detected in sediments and rocks, and it dissolves in anoxic groundwater (Hem, 1985; Lin and Puls, 2000). Chronic ingestion of arsenic at concentrations greater than the EPA MCL of 10 µg/L has been linked to increased risk of lung, bladder, skin, liver, kidney, and prostate cancer (U.S. Environmental Protection Agency, 2018; table 3). Arsenic concentrations exceeded the MCL in about 24 percent (11 of 45) of the monitoring wells, and most locations had concentrations similar to what was measured in 2010, with slight increases in 17–ALG–D from 16.1 µg/L in 2010 to 20.7 µg/L in 2020 and in 1–CHE–D from 62 µg/L in 2010 to 70.3 µg/L in 2020 (fig. 6). The screen interval at monitoring well 1–CHE–D is open to the top of the limestone bedrock aquifer and had low dissolved oxygen (0.2 mg/L). The geochemically reducing conditions may increase the dissolution of arsenic-bearing minerals within the limestone aquifer (Renard and others, 2015). MARN–10–03 (10.1 µg/L) and MARN–10–04 (16.3 µg/L) were sampled in 2020 but were not available to sample in 2010; both wells had detections of arsenic greater than the MCL. Detections for arsenic at the two surface-water sites that were sampled did not exceed the MCL for arsenic.
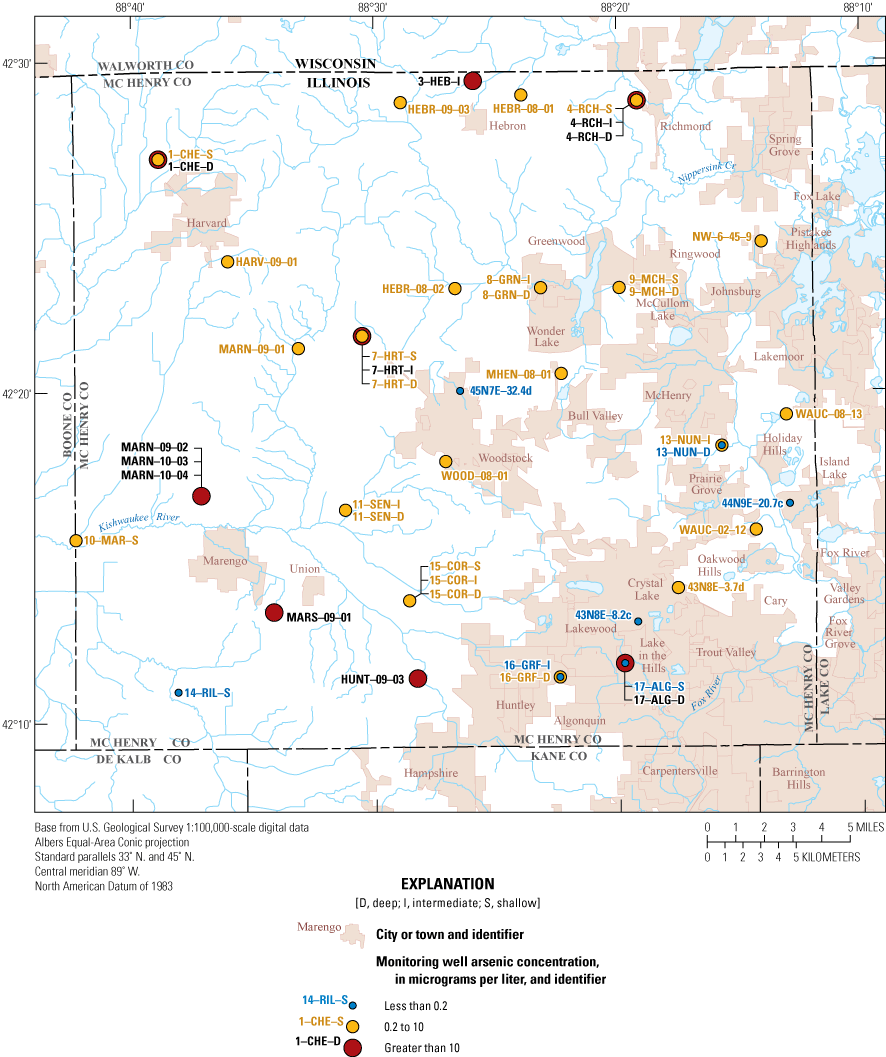
Distribution of arsenic concentrations in the McHenry County Groundwater Monitoring Network wells and the National Water-Quality Assessment monitoring wells, McHenry County, Illinois, 2020.
Iron concentrations in groundwater ranged from less than 10 to 3,478 µg/L with a median of 1,739 µg/L. Groundwater in McHenry County has naturally elevated iron from the dissolution of glacial aquifer material and is consistent with previous water-quality investigations and this 2020 decadal update (Groschen and others, 2008; Gahala, 2017). Iron has an SMCL of 300 µg/L, and concentrations exceeding the SMCL can cause staining of household fixtures and laundry (National Ground Water Association, 2010). Concentrations greater than 1,800 µg/L can impart a metallic taste in water (Fetter, 1980, p. 355). A total of 87 percent (39 of 45 wells) exceeded the 300-µg/L SMCL, and 40 percent (18 of 45 wells) exceeded the 1,800-µg/L concentration (fig. 7). Iron in surface-water samples was less than the reporting level of 10 µg/L.
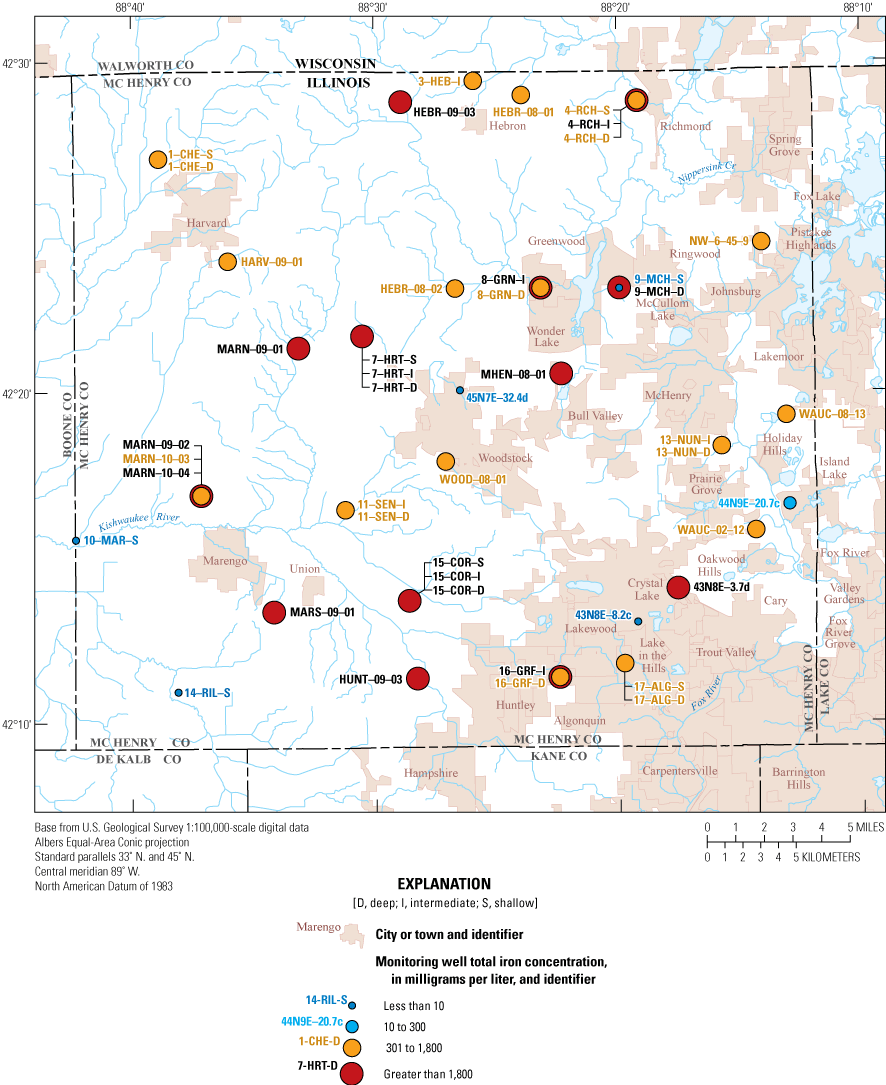
Distribution of iron concentrations in the McHenry County Groundwater Monitoring Network wells and the National Water-Quality Assessment monitoring wells, McHenry County, Illinois, 2020.
Manganese ranged from 0.5 to 740 µg/L with a median of 25.6 µg/L (table 6). Manganese naturally occurs in aquifer sediments but also can have anthropogenic sources such as industrial effluent, acid-mine drainage, sewage, and landfill leachate (Nádaská and others, 2010; World Health Organization, 2011). Long-term exposure to DWA exceedances of manganese can cause toxicity to the nervous system (World Health Organization, 2011). Manganese has a DWA of 300 µg/L (exceeded at one well) and an SMCL of 50 µg/L. Similar to iron, manganese is naturally abundant in groundwater in McHenry County because of dissolution of manganese-oxide coatings on glacial aquifer material (Groschen and others, 2008). Concentrations greater than the SMCL can cause dark brown or black staining on household fixtures and laundry. About 29 percent (13 of 45 wells) exceeded the SMCL, and the highest concentration was nearly 15 times the SMCL (740 µg/L at 10–MAR–S; fig. 8; table 3). Manganese concentrations in surface water did not exceed the aesthetic or health benchmarks.
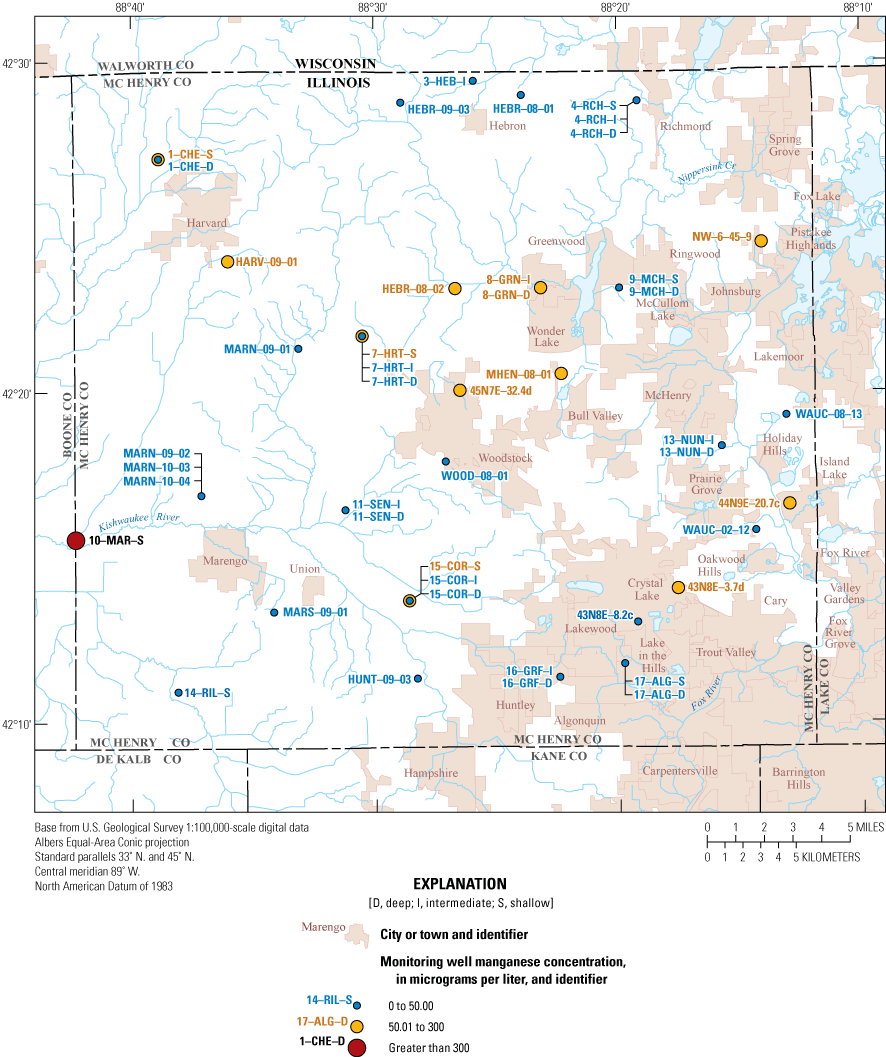
Distribution of manganese concentrations in the McHenry County Groundwater Monitoring Network wells and the National Water-Quality Assessment monitoring wells, McHenry County, Illinois, 2020.
Nutrients
Nitrogen (nitrate plus nitrite as nitrogen, hereafter referred to as “nitrate”) and phosphorus naturally occur in the environment but also can come from anthropogenic sources such as fertilizers, animal manure, and sewage (Gahala, 2017). Anthropogenic sources of nutrients, such as fertilizers, have increased the concentrations of nitrate in many streams and aquifers (U.S. Geological Survey, 1999; Dubrovsky and others, 2010; Domagalski and Johnson, 2012). In total, 8 of 45 wells, or about 18 percent of the sampled monitoring wells, had detections for nitrate (0.08 to 5.33 mg/L) and all were shallow (less than a 50-ft depth) (table 7). Nitrite ranged from less than the reporting level of 0.001 to 0.054 mg/L with a median of less than 0.001 mg/L as nitrogen. Orthophosphate ranged from less than the reporting level of 0.004 to 0.141 mg/L as phosphorus with a median of 0.010 mg/L as phosphorus. Ammonia ranged from less than 0.01 to 2.04 mg/L as nitrogen with a median of 0.34 mg/L as nitrogen. Nippersink Creek and Fox River were the two surface-water sites sampled for nutrients (table 7). Nitrate, nitrite, and orthophosphate were detected at Nippersink Creek, but the concentration of ammonia was less than the reporting level of 0.01 mg/L as nitrogen.
Table 7.
Results for concentrations of nutrients from samples collected from the McHenry County Groundwater Monitoring Network wells, 4 National Water-Quality Assessment wells, and 2 surface-water sites in McHenry County, Illinois, 2020.[Data available from U.S. Geological Survey, 2020b. mg/L, milligram per liter; N, nitrogen; NO3, nitrate; NO2, nitrite; P, phosphorus; <, less than; U, nondetect; M, detect; IL, Illinois; —, no data]
No detections of nitrate exceeded the MCL of 10 mg/L in 2020. Nitrate concentrations ranged from less than the reporting level to 5.33 mg/L as nitrogen. Although no nitrate samples exceeded the MCL of 10 mg/L, a few sites did indicate slight increases. Well 17–ALG–S increased from 0.71 mg/L as nitrogen in 2010 to 1.57 mg/L as nitrogen in 2020 and 10–MAR–S increased from 0.23 mg/L as nitrogen in 2010 to 1.52 mg/L as nitrogen in 2020. Nitrite concentrations ranged from less than the reporting level of 0.001 to 0.054 mg/L as nitrogen and did not exceed the MCL of 1 mg/L for the health-based benchmark in any samples. Nitrite is unstable in aerated water and is generally considered to be an indicator of contamination from sewage or organic waste (Hem, 1985) if present. Results for all nutrients are summarized in table 7.
A dissolved oxygen concentration of 2.0 mg/L is a common threshold for denitrification to exist; below this concentration, denitrification becomes favorable (McCallum and others, 2008). In all but one well, detections of nitrate were coincident with dissolved oxygen concentrations greater than 2.0 mg/L, and most of these wells were shallow wells (20–48 ft). The one exception is well 17–ALG–S, where the dissolved oxygen concentration was 0.4 mg/L and the nitrate concentration of 1.57 mg/L as nitrogen was relatively high. One potential explanation for the lower dissolved oxygen and elevated nitrate concentration may be that groundwater can move quickly through sand and gravel aquifer materials along a preferential flow path or that dissolved oxygen decreases at a faster rate than denitrification.
Contaminants of Emerging Concern
CECs are pharmaceuticals, detergents, natural and synthetic hormones, pesticides, and other chemicals such as WICs, plastic components, surfactant metabolites, and fire retardants (U.S. Environmental Protection Agency, 2009b; Tomasek and others, 2012) that are not typically treated for in wastewater-treatment plants. CECs that may be removed at wastewater-treatment plants are incidental to biological treatment and disinfection processes (U.S. Environmental Protection Agency, 2009b; U.S. Geological Survey, 2018). The release of CECs to the environment is a growing concern because of the potential to cause adverse effects in humans and other nontargeted organisms (Kolpin and others, 2002), and the use of pharmaceuticals has increased by 2.8 percent since 2015 (Martin and others, 2019; Centers for Disease Control and Prevention, 2021). The most commonly prescribed medications include analgesics, antihyperlipidemic agents, and dermatological agents (Centers for Disease Control and Prevention, 2021). WICs are a general category for anthropogenically derived chemicals that include some pharmaceuticals, pesticides, and some volatile organic compounds all quantified in parts per billion (micrograms per liter). Pharmaceuticals were analyzed in parts per trillion (nanograms per liter) and include the most commonly used prescription drugs for antibiotics, opioids, diabetes, anti-inflammatories, and high blood pressure and cholesterol (statins) medication. Atrazine is also used as an antihistamine and is referred to in the results as “pharmaceutical atrazine,” and thiabendazole is an antiworm medication (Drugs.com, 2021; WebMD, 2021).
In 2020, a subset consisting of 12 monitoring wells and 3 surface-water sites was sampled and analyzed for CECs, including pharmaceuticals, pesticides, and WICs. Chloride-bromide ratio analyses completed in Gahala (2017) identified wells that plotted near the sewage mixing curve or indicated a mix of sewage and dilute road salt. Monitoring wells with chloride-bromide ratio results in 2010 that plotted near the sewage mixing curve or mixture of sewage and dilute road salt were HEBR–09–03, 15–COR–I, 10–MAR–S, 9–MCH–S, MARN–09–01, 8–GRN–I, HARV–09–01, 4–RCH–S, and HEBR–08–02. Designated background wells were selected based on their chloride-bromide ratio plotting near high road salt mixing curves (7–HRT–S) or within the dilute groundwater range or with no clear proximity towards a particular mixing curve (8–GRN–D and WOOD–08–01).
A total of 172 CECs were analyzed for in the groundwater monitoring wells, and only 5 percent of the CECs were detected in either low or estimated concentrations, indicating a lack of widespread presence of CECs in the shallow groundwater resource and supporting the overall good quality of the shallow groundwater resource (table 8). A total of 9 CECs were detected at low concentrations in groundwater samples from 8 of the 12 (about 66 percent) monitoring wells (fig. 9; table 8); however, 3 of the 9 CECs also were detected in at least 1 blank QAQC sample (appendix 1, table 1.2; Gahala and Gruhn, 2022). These CECs are formatted in bold italics and flagged as a potential but not verified detection (table 8). The most common uses of the pharmaceuticals are provided in the parentheses in table 8 and were obtained from searching the database available at https://www.drugs.com/ (Drugs.com, 2021). Of the 111 pharmaceuticals analyzed, 2 (2 percent) pharmaceuticals were detected in groundwater samples; caffeine was detected in 1 well and nicotine was detected in 6 wells (table 8). Of the 61 WICs analyzed, 7 (about 11 percent) were detected in five groundwater samples, 10–MAR–S and 9–MCH–S had detections of 2 WICs. A total of three WIC detections are flagged because they also were detected in at least one blank QAQC sample (appendix 1, table 1.2; Gahala and Gruhn, 2022). N,N-Diethyl-m-toluamide (commonly known as DEET) was detected in every groundwater sample and surface-water sample, including all the QAQC samples (appendix 1, table 1.2; Gahala and Gruhn, 2022). This compound was removed from the results tables and discussions because of its ubiquitous detection and questionable source. The low level and often estimated concentrations not only indicate the vulnerability of groundwater with respect to sewage and septic effluents but also highlight the potential capacity of the aquifer to degrade or mitigate some of the detected CECs.
Table 8.
Concentrations of pharmaceuticals and wastewater indicator compounds detected in samples collected from selected wells within the McHenry County Groundwater Monitoring Network and three surface-water sites in McHenry County, Illinois, 2020.[Data available from U.S. Geological Survey, 2020b. Bold values are detections greater than the detection or reporting level. Bold italic values had a detection in one or more blank quality assurance and quality control samples. CEC, contaminant of emerging concern; Pharm, pharmaceuticals; ng/L, nanogram per liter; <, less than the reporting level; E, estimated; WIC, wastewater indicator compound; µg/L, microgram per liter; HHCB, Hexahydrohexamethyl cyclopentabenzopyran; TBEP, Tris(2-butoxyethyl) phosphate]
Common use of pharmaceutical from Drugs.com, 2021.
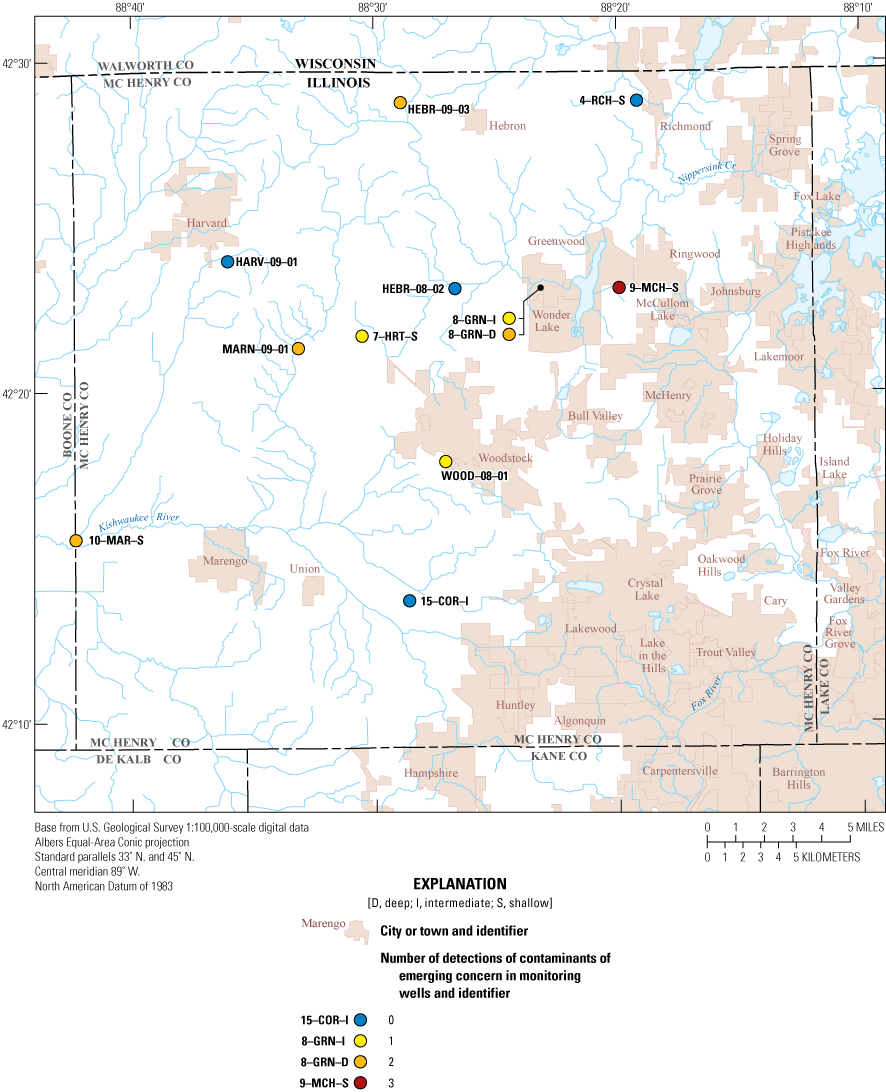
Number of detections for pharmaceuticals, pesticides, and wastewater indicator compounds in the McHenry County Groundwater Monitoring Network wells and four National Water-Quality Assessment monitoring wells, McHenry County, Illinois, 2020.
The surface-water sampling and analysis of CECs provide a general indication of the presence of CECs at locations downstream from multiple or specific wastewater-treatment discharges. The results are based on a single sample collection at three surface-water sites and do not constitute a robust analysis of the sources, range in concentrations, seasonality, and persistence. Of the 172 CECs analyzed, 35 (about 20 percent) CECs were detected in at least 1 surface-water sample and 18 were detected at all 3 surface-water sites. Of the 111 pharmaceuticals analyzed, 20 were detected in the surface-water samples, including the pharmaceutical atrazine. Of the 61 WICs analyzed, 15 were detected in surface-water samples, including the pesticide atrazine (table 8). Surface waters indicated the highest concentrations and most frequent detection of CECs. These locations were selected based on proximity to wastewater-treatment plant effluent, and the detections highlight the limits of the treatment systems.
The analytical methods for pharmaceutical compounds, in nanograms per liter, and WICs, in micrograms per liter, naturally create high variability in the quantified results of detections. For detailed discussion of the groundwater QAQC results, refer to appendix 1. The groundwater results discussed consider only the constituents that were detected at low or estimated quantities in the sample or replicate and omit consideration or discussion of constituents detected in the blank samples. No equipment blanks were collected for the surface-water sample collection. Surface-water results are considered acceptable for discussion of general presence and frequency of detections among the three samples.
Pharmaceuticals are used by individuals for health reasons and include over-the-counter medication such as aspirin and pseudoephedrine, and medications prescribed by a physician such as Lipitor, albuterol, amoxicillin, and many others (U.S. Environmental Protection Agency, 2009b). Most ingested pharmaceuticals are not fully metabolized, so parts are excreted in human waste. Metabolized and unmetabolized pharmaceuticals are discharged in domestic sewage (U.S. Environmental Protection Agency, 2009b; U.S. Geological Survey, 2021a, b). Carbamazepine is an anticonvulsant used to prevent and control seizures (Drugs.com, 2021) and was detected (2.9 nanograms per liter [ng/L]) in the replicate sample from 9–MCH–S, but was not detected in the primary environmental sample (appendix 1, table 1.2; Gahala and Gruhn, 2022). Caffeine was detected in HEBR–09–03, estimated at 5.5 ng/L. Pharmaceutical atrazine was detected in the replicate of 10–MAR–S at 5.03 ng/L (appendix 1, table 1.2; Gahala and Gruhn, 2022) but was not detected in the environmental sample at 10–MAR–S. Nicotine was detected at low and estimated concentrations in 50 percent (6 out of 12) of the groundwater samples. The number of CEC detections in the MCGMN are shown in figure 9.
Fox River and Kishwaukee River had the greatest number of pharmaceuticals detected (18 in each), whereas Nippersink Creek had 16 pharmaceutical detections. The highest concentrations detected in the three surface-water sites were for methylbenzotriazole, hexamethylenetetramine, fexofenadine, metformin, sulfamethoxazole, carbamazepine, and caffeine. The Kishwaukee River had the higher detections of lidocaine, methocarbamol, tramadol, metoprolol, venlafaxine, famotidine, and desvenlafaxine than the other two surface water sites. Nippersink Creek had higher concentrations of atrazine, cotinine, and acetaminophen than the Fox River or Kishwaukee River. Of the 13 pharmaceuticals detected in all 3 surface-water samples, concentrations were generally highest in Fox River and Kishwaukee River.
Wastewater indicators are chemical compounds that indicate the presence of domestic and industrial waste in water sources such as streams and aquifers (U.S. Geological Survey, 2021b). Domestic WICs include caffeine and cotinine (a byproduct of nicotine metabolism in the liver; Bradley and others, 2007). Industrial wastewater indicators include compounds used in plastic components, surfactant metabolites, fire retardants, and many others (Tomasek and others, 2012). Several WICs were detected in the groundwater samples from the MCGMN, and many more were detected in surface-water samples from Nippersink Creek, Fox River, and the Kishwaukee River. The following paragraphs discuss the results of WICs that are classified as “organic other” and do not fall under the pharmaceutical or pesticide/herbicide categories discussed in the previous sections.
FYROL FR 2 (0.05 µg/L) was detected in the groundwater at 10–MAR–S and is a common additive flame retardant in commercially used flexible and rigid polyurethane foams (Chemical Book, 2021). Indole (0.08 µg/L) was detected in the groundwater at 8–GRN–D and has many uses (cleaning agent, food additive, veterinary drug, fragrance, and more; PubChem, 2021a). A common food additive and fragrance (PubChem, 2021b), 2,6-Dimethylnapththalene (0.01 µg/L), was detected in the groundwater at HEBR–09–03.
Detections of WICs in surface water were most frequent and at greater concentrations in the Fox River but were generally ubiquitous in all three surface-water locations (table 8). WICs (organics/other, not including pesticides) detected in samples from all three surface-water locations include β-sitosterol (cosmetics, detergent, veterinary drug; PubChem, 2021c) and diethyl phthalate (plasticizer, solvent, household products, and more; PubChem, 2021d). No endocrine disrupting compounds such as 4-nonylphenols were detected at concentrations greater than reporting level in any of the surface-water samples. Endocrine disrupting compounds are known to cause various hormone and reproductive effects on aquatic life (U.S. Environmental Protection Agency, 2022b).
Pesticides are chemicals used to prevent, destroy, or repel living organisms from where they are not wanted (U.S. Environmental Protection Agency, 2009b). Pesticides are frequently used to increase crop yields and improve the quality of the crops; however, they can be transported to groundwater and surface water (U.S. Geological Survey, 1999, 2021a). Results for pesticide analyses are presented in table 8.
Four pesticides (prometon, atrazine, metolachlor, and pentachlorophenol) were detected in the water samples collected in 2020. Prometon is an herbicide used to control the emergence of most annual and many perennial broadleaf weeds and grasses in nonagricultural areas (U.S. Environmental Protection Agency, 1990). Prometon was detected in 9–MCH–S (estimated 0.04 ng/L; table 8) and also was detected in the associated replicate sample (0.04 ng/L; table 1.2). No detections of prometon were in the surface-water samples.
Atrazine is a common and widely used herbicide that can be applied before and after planting to control broadleaf and grassy weeds (U.S. Environmental Protection Agency, 2021a). Atrazine is used primarily in agriculture, but to a lesser extent, it is sometimes used on residential lawns and golf courses (U.S. Environmental Protection Agency, 2021a). As indicated in table 8, atrazine also can be used as antianxiety medication; therefore, atrazine has two results, the pharmaceutical method analyzed concentrations in parts per trillion (nanograms per liter), and the WIC analysis measured concentrations in parts per billion (micrograms per liter). Atrazine was not detected in any environmental samples from groundwater wells, although it was detected in the replicate sample for 9–MCH–S (3.29 µg/L) and the replicate sample for 10–MAR–S at the parts per trillion concentration of 5.03 ng/L (table 1.2). This inconsistency in detection between the parts per billion and parts per trillion concentrations and between the environmental and replicate samples highlights the variability of detections at these low levels with the laboratory methods applied as described in the “Methods of Study” section and detailed in the cited references.
All three surface-water sites had detections for atrazine (table 8). Atrazine was highest in Nippersink Creek with a concentration of 299 ng/L, as detected with the pharmaceutical method, and also was detected at 0.31 µg/L (315 ng/L) with the WIC analytical method. Consistent concentrations of atrazine between the pharmaceutical analysis and WIC analytical method also were noted for the Fox River and Kishwaukee River. Metolachlor is a broad-spectrum herbicide used for general weed control in numerous agricultural crops, lawns and turf, ornamental plants, trees, shrubs, right of ways, fence and hedge rows, and in forestry (U.S. Environmental Protection Agency, 1995). Metolachlor was not detected in any of the groundwater wells, although it was detected at all surface-water sites. One surface-water site (Fox River) had a detection for pentachlorophenol (estimated 0.13 μg/L), but no other detections of this pesticide were in surface water or groundwater.
Suitability of Water for Drinking
The largest concern for causing negative effects to the quality of groundwater as a source of drinking water is for arsenic, iron, manganese, and dissolved solids, which are naturally occurring in the sand and gravel aquifers in McHenry County (table 3). The MCL of 10 µg/L for arsenic was exceeded in about 24 percent of the wells, and chronic ingestion of arsenic in exceedance of the MCL has been linked to increased risk of lung, bladder, skin, liver, kidney, and prostate cancer (U.S. Environmental Protection Agency, 2018; table 3). Manganese, which can cause toxicity to the nervous system, has a health-based drinking water advisory of 300 µg/L, and that was exceeded at one location (U.S. Environmental Protection Agency, 2018; table 3). Aesthetically, the SMCL of 500 mg/L for dissolved solids was exceeded at 13 locations (about 29 percent). Dissolved solids in exceedance of the SMCL can cause objectionable taste and, at concentrations greater than 1,000 mg/L, can have laxative effects (U.S. Environmental Protection Agency, 2018; table 3). Two sites had dissolved solids concentrations that exceeded 1,000 mg/L (4–RCH–S and 43N8E–3.7d) (table 5). Aside from health concerns, the main concern with most of these constituents is that treatment would be needed to prevent damage to infrastructure and staining of household appliances and laundry. Another major concern for drinking water is nutrient concentrations, especially nitrate and nitrite, which have an MCL of 10 mg/L and 1 mg/L, respectively. No exceedances of the MCL were measured for either nitrate or nitrite. In general, the groundwater in McHenry County is suitable for drinking water, and some of the aesthetic issues could be mitigated with home water-treatment systems such as water softeners. The concentrations of many of these constituents could also be reduced with an at-home water-treatment system such as a carbon filtration, ion-exchange systems, and reverse-osmosis systems (Thomas and Eckberg, 2015).
The implications of low concentrations of CECs in surface water on drinking water and aquatic life are unknown, and it is important to reiterate that these results are a single sample at three sites and do not constitute a robust understanding of the distribution and persistence of these CECs. These pharmaceuticals, pesticides, and low concentrations of WICs are potentially ingested by consumers of stream-sourced drinking water and aquatic biota within the streams (Wilson and others, 2011). In a study by Benson and others (2017), the potential long-term toxicity of CECs to humans for the most frequently detected and highest concentrations of WICs and pharmaceuticals were quantified with a risk-assessment analysis. The study concluded that the exposure to these CECs is not likely to pose a public health concern; however, additional toxicity data would be helpful to improve the calculated risks (Benson and others, 2017). Also, studies have determined that many of these CECs may degrade naturally in the streams (Bradley and others, 2007) whereas others persist miles downstream (Barber and others, 2013), including some of the contaminants detected in this study (sulfamethoxazole and carbamazepine). The persistence, seasonality, sources, and range in concentrations and effects of CECs in surface water were not investigated as part of this study. Frequent testing by water-treatment facilities provides the data on exposure levels and baseline understanding for potential effects.
Comparison to 2010 Water-Quality Results
The following sections compare the 2020 water-quality results to the baseline results of 2010. Differences in water quality on the decadal time scale were evaluated with the WSR test (Helsel, 2012) to identify statistically significant differences. Shifts in chloride to bromide ratio plots also are evaluated to identify potential changes in water quality.
Statistical Comparison
The results of the WSR to assess changes in the water-quality results between 2010 and 2020 indicated a few statistically significant differences between the two sampling events (table 9). Differences were statistically significant among all wells for concentrations of aluminum, ammonia, arsenic, barium, bromide, calcium, magnesium, molybdenum, orthophosphate, silica, specific conductance, sulfate, and dissolved solids. Statistically significant differences (p-value of less than [<] 0.05) were calculated among shallow (20.3–58.7 ft below ground surface), intermediate (62.3–120.6 ft below ground surface), or deep (139–344.4 ft below ground surface) aquifer groups between the 2010 and 2020 water-quality results. Statistically significant differences were noted for aluminum (deep), arsenic (shallow), barium (deep and intermediate), calcium (deep and intermediate), magnesium (deep and intermediate), manganese (intermediate), molybdenum (deep and intermediate), orthophosphate (deep and shallow), and silica (deep and intermediate) (table 9). In figure 10, a change plot shows pairs of linked points that are the two samples collected in 2010 and 2020 for a given well. If both samples for a given well were censored, the lower value was recensored to the higher value. If only one of the samples was uncensored but less than the other sample’s reporting level, the uncensored value was censored to match the censored sample. These “within pair” recensored values were only computed and used for plotting; they were not used in the statistical analyses. Censored values are indicated with open circles. Whether the change between 2010 and 2020 was downward, upward, or the same (common when both values were censored) is indicated by the color of the points and line.
Table 9.
Summary statistics (minimum, maximum, and median) for selected constituents, results of the Wilcoxon signed-rank test for groundwater-quality changes between 2010 and 2020 for uncensored data and the paired Prentice-Wilcoxon test for uncensored data, and evaluation of differences between the 2010 and 2020 aquifer-depth groups of shallow, intermediate, and deep parts of the sand and gravel aquifers in McHenry County, Illinois.[Bold denotes statistically significant; p-value, probability value; µg/L, microgram per liter; <, less than reporting level; PPW, paired Prentice-Wilcoxon test; —, no data or not applicable; mg/L, milligram per liter; N, nitrogen; WSR, Wilcoxon signed-rank test; µS/cm, microsiemens per centimeter at 25 degrees Celsius]
Increases generally were detected in the intermediate and deep parts of the sand and gravel aquifer, and decreases were generally detected in the shallow part of the sand and gravel aquifer (fig. 10). The mixed distribution of increases and decreases among the various constituents and aquifer-depth groups could be reflecting dissolution and mobility of some of the redox sensitive constituents and dilution of some constituents in the shallow aquifer depths. These differences may be attributed to a combination of stable population of the past decade (2010–20), to land-use management practices, or potentially to the recent wet years of 2017 through 2019 causing a dilution of the major ions in the shallow parts of the aquifer.
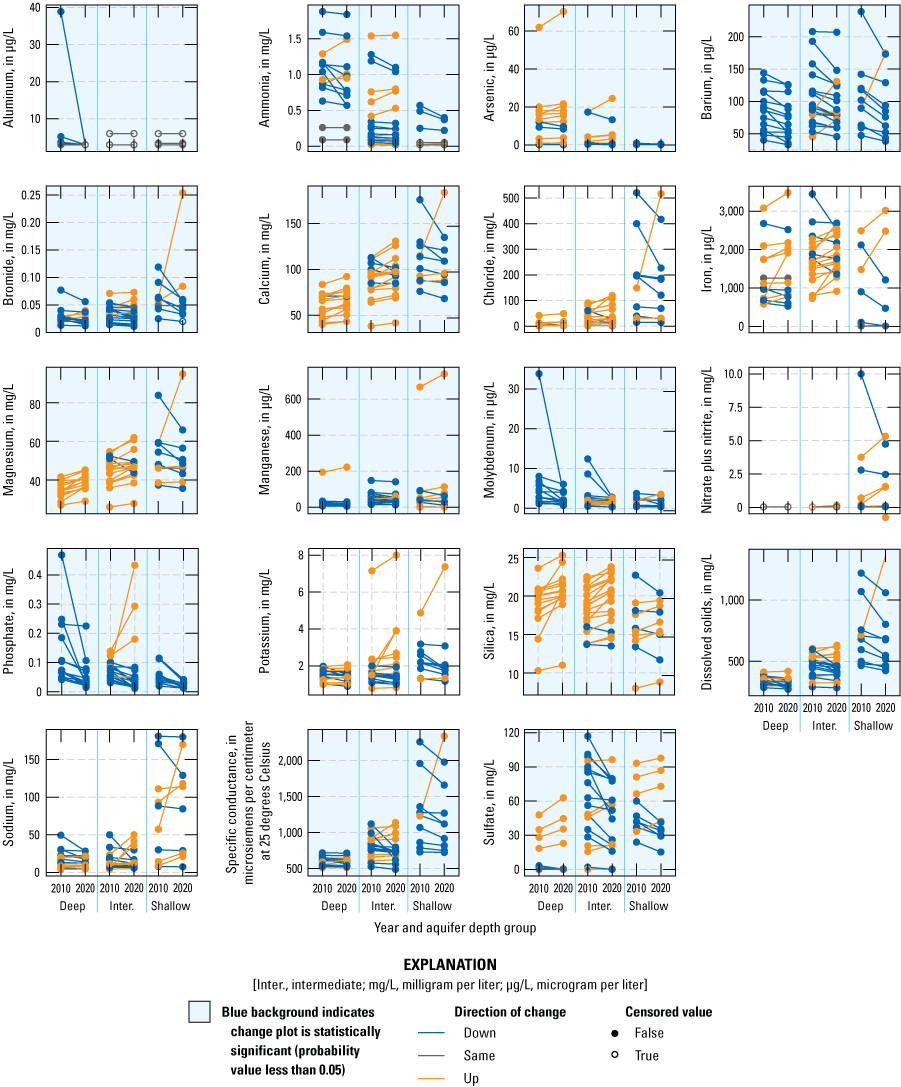
Differences in the shallow, intermediate, and deep parts of the sand and gravel aquifers for concentrations of selected constituents between 2010 and 2020 for water-quality samples in McHenry County, Illinois.
The descriptive statistics and a trilinear Piper diagram (Piper, 1944; fig. 11A, B) also were reviewed to identify relative changes in the range and average of selected constituents of interest. A slight shift was seen in the anions in the Haeger-Beverly Unit (shallow aquifer) between 2010 and 2020 with a decrease in sulfate and chloride (fig. 11A). The trilinear Piper diagram for all other wells in the network indicates overall consistency in concentrations of major ions from 2010 to 2020 (fig. 11B). The minimum, median, and maximum for selected constituents for 2010 and 2020 are listed in table 9. Only calcium, magnesium, and silica indicated a slight increase in minimum, median, and maximum concentrations.
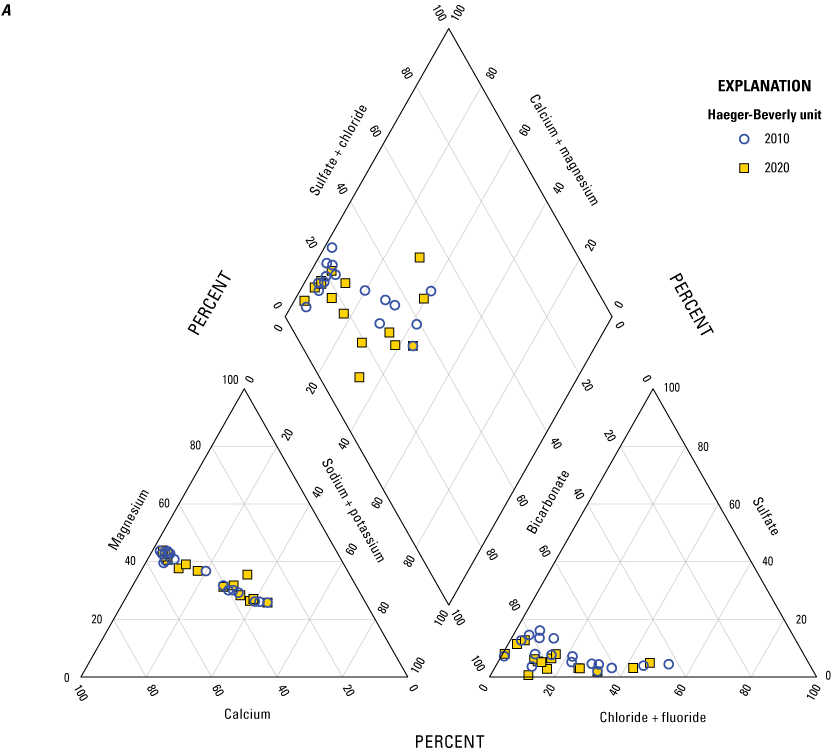

Major ions in wells from 2010 and 2020 in McHenry County, Illinois. A, wells completed in the Haeger-Beverly Unit; B, all other wells in the network.
Comparison of Chloride to Bromide Ratios
The methods for chloride-bromide ratio analyses used in Gahala (2017) were repeated for the 2020 samples. Bromide is a conservative ion (similar to chloride) that can be deposited from precipitation and infiltrate into groundwater (Davis and others, 1998) or dissolved from sedimentary rock such as carbonates, shale, and evaporites (Salameh and others, 2016). Concentrations of bromide in groundwater are usually 40–8,000 times less than concentrations of chloride, so small changes in the mass of bromide produce large variations in the ratios of chloride to bromide, which can help trace the source of the chloride (Davis and others, 1998; Gahala, 2017). The 2020 sample results were used, and the chloride-bromide ratios were plotted on the same graph as the source binary mixing curves to evaluate sources affecting water quality and assess any changes in water quality at each monitoring well since 2010 (figs. 12 and 13). The proximity of the plotted chloride-bromide ratio to the source-mixing curve indicates the potential source for the chloride in the surrounding groundwater. Chloride-bromide ratios were estimated for monitoring wells with bromide concentrations less than the reporting level (<0.020 mg/L) by halving the reporting level (0.01) to estimate a point on the curve. Plot points for 17–ALG–S, 7–HRT–I, 4–RCH–I, and 45N7E–32.4d are estimated and do not plot on any curves but plot to the left of the curves.
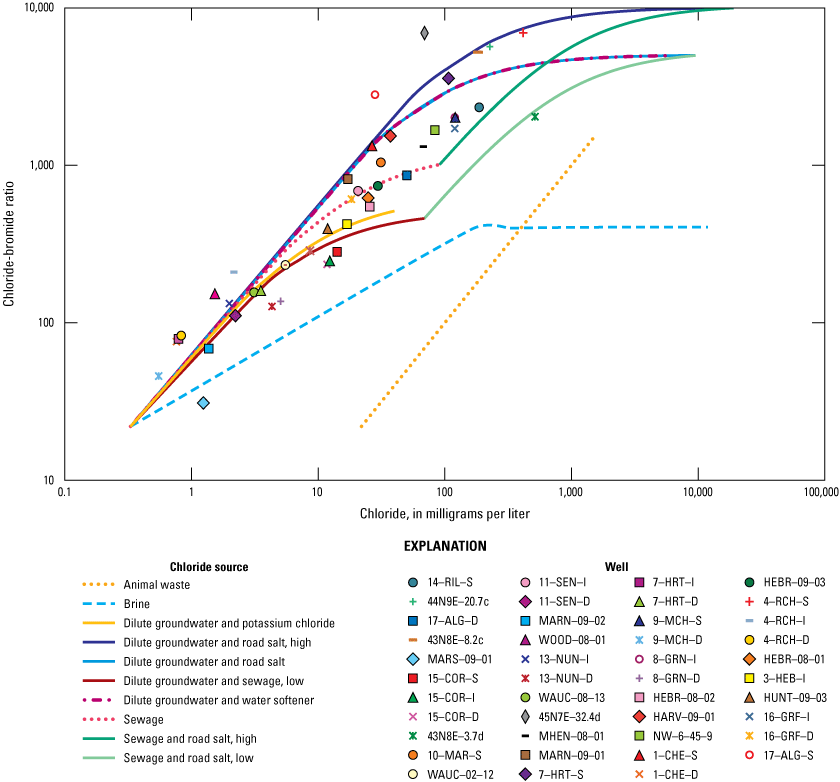
Chloride-bromide ratio plotted against chloride concentrations in the McHenry County Groundwater Monitoring Network wells and the National Water-Quality Assessment monitoring wells, McHenry County, Illinois, 2020.
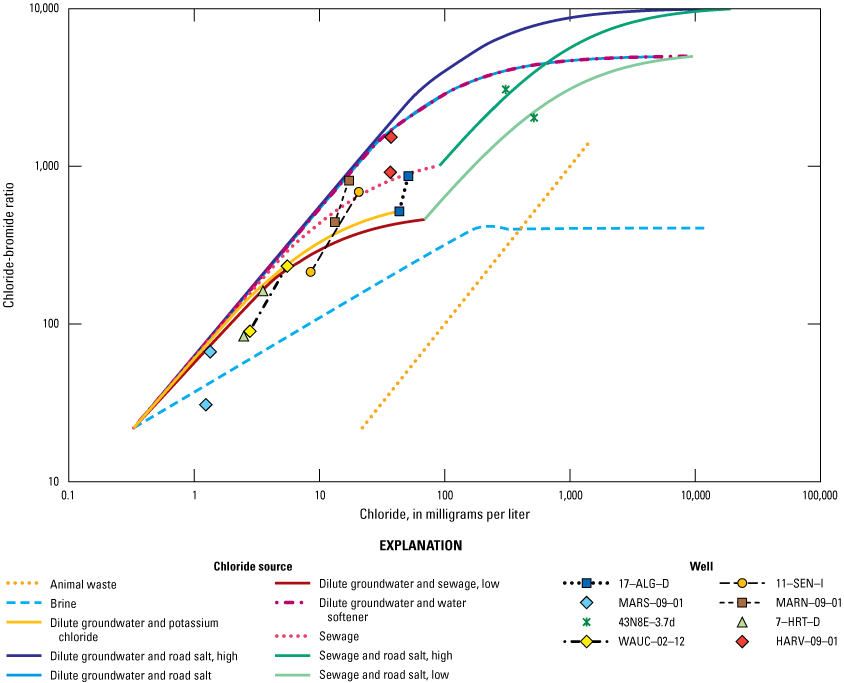
Chloride-bromide ratio plotted against chloride concentration for wells that show shifts between 2010 and 2020 in the McHenry County Groundwater Monitoring Network wells and the National Water-Quality Assessment monitoring wells, McHenry County, Illinois, 2020.
In general, the 2020 chloride-bromide ratios plotted similarly to where they plotted in 2010, indicating the source of the chloride had not changed. CECs identified in 10–MAR–S, MARN–09–01, 9–MCH–S, 8–GRN–D, and HEBR–09–03 are consistent with the source water identified in the chloride-bromide ratio analysis. A few locations did indicate shifts toward a specific binary-mixing curve, and these locations are discussed in this section (fig. 13). The chloride-bromide ratio analysis for monitoring well 11–SEN–I indicated a shift from the dilute groundwater and sewage (low) mixing curve to plotting close to the sewage mixing curve (fig. 13). Monitoring wells 11–SEN–I and 11–SEN–D are near a septic field for a municipal building. Chloride and sulfate concentrations slightly increased since 2010 in 11–SEN–I. Increased sulfate concentrations could be indicative of increased microbial activity, possibly from sewage (table 5). Chloride concentrations increased at 11–SEN–I from 8.53 to 20.7 mg/L, and sulfate concentrations increased from 45.9 to 56.8 mg/L (Gahala, 2017; table 5). The shift more towards the sewage mixing curve may be indicative of ongoing and potentially increasing septic-field effect at this location.
Monitoring well HARV–09–01 shifted from near the sewage source curve in 2010 towards the dilute groundwater and water-softener curve in 2020. Other constituents, such as alkalinity, pH, nutrients, major ions, and metals, were reviewed to observe if any changes were consistent with this change. In general, no differences were statistically significant despite there being slight decreases in nutrient concentrations and slight increases in iron concentrations. This well also was sampled and analyzed for CECs in 2020 because it previously plotted near the sewage mixing curve. The results of the CEC analyses were all less than the reporting level, indicating sewage is not likely affecting the groundwater quality at HARV–09–01. The shift to another source curve could be highlighting the uncertainty of the chloride-bromide ratio analysis, or the source of the groundwater at this location could be from a mixture of sources; future sampling events may clarify this result.
The chloride-bromide ratios for 17–ALG–D shifted from plotting on the dilute groundwater and potassium chloride curve to plotting on the sewage curve. The surroundings of this well are split between agriculture and urban land (Gahala, 2017). A few other constituents that either increased or decreased (not significantly) could explain the shift from the dilute groundwater and potassium chloride curve to the sewage curve. Manganese nearly doubled in concentrations from 6 to 11.4 mg/L, magnesium increased from 28.6 to 36.4 mg/L, sulfate decreased from 3.28 to 0.24 mg/L, and sodium decreased from 49.4 to 28.3 mg/L (Gahala, 2017; tables 5 and 6). Nutrients such as ammonia and orthophosphate also decreased. This well is a candidate for future sampling for CECs to monitor for any continued changes or effects.
NAWQA monitoring well 43N8E–3.7d shifted from between the dilute groundwater and water softener and the sewage and road salt (high) curves in 2010 to nearly on the curve for the sewage and road salt (low) mixing curve in 2020. Chloride concentrations increased from 149 mg/L in 2010 to 517 mg/L in 2020, sodium concentrations increased from 57.3 mg/L in 2010 to 170 mg/L in 2020, and bromide concentrations increased from 0.061 mg/L in 2010 to 0.254 mg/L in 2020 (Gahala, 2017; table 5). Other constituents also have changed with increases in sodium, iron, manganese, magnesium, potassium, barium, and dissolved solids, all of which indicate a potential effect on water quality and subsequent shift in geochemistry. This well is in an urban area and could receive anthropogenic effects from leaky sewers and road salt infiltration. Previous investigations that analyzed for volatile organic compounds did not indicate detectable levels of contaminants.
MARN–09–01 shifted from between the curves of sewage and dilute groundwater and potassium chloride in 2010 to nearly on the curve for the dilute groundwater and water-softener curve in 2020. Few constituents indicated increases in concentrations except for chloride, calcium, sulfate, and iron, which were slight increases. The land use surrounding MARN–09–01 is agriculture and urban (Gahala, 2017). This well also was selected for analysis of CECs because the orthophosphate detected in this well in 2010 was the highest of any of the other wells sampled that year. The 2020 orthophosphate results indicated a reduction from 0.152 mg/L in 2010 to 0.035 mg/L in 2020 (table 7). It was previously concluded that this well may have been under the effect of a previous septic field from a former house near the monitoring well (Gahala, 2017). The CEC analysis detected nicotine (estimated 35.2 ng/L; table 8). The compound triethyl citrate was detected, but this compound was detected in the equipment blank (associated with 10–MAR–S); therefore, it is a suspected detection but not confirmed (appendix 1, table 1.2; Gahala and Gruhn, 2022). Nicotine is a likely detection because this constituent was not detected in field blanks or equipment blanks. The source of the chloride in 2010 plotted near the sewage mixing curve, but in 2020, the source is indicated as being affected by water softeners. In either case, an anthropogenic source of the chloride is implicated.
Summary
McHenry County, Illinois, obtains most its drinking water from the shallow sand and gravel aquifers (groundwater). To evaluate this groundwater resource, the U.S. Geological Survey collected water-quality samples from 41 of the 42 monitoring wells in the McHenry County Groundwater Monitoring Network, 4 monitoring wells from the National Water-Quality Assessment program, and 2 surface-water sites for analysis of nutrients, major ions, and trace metals. Additionally, a subset of 12 monitoring wells and 3 surface-water sites was sampled and analyzed for contaminants of emerging concern (CECs). The 2020 results were summarized and compared to the 2010 water-quality data to identify any differences in the water quality of the sand and gravel aquifers in McHenry County, Illinois, which is the primary source of drinking water.
Health-based benchmarks were exceeded for arsenic (about 24 percent; 11 of 45 monitoring wells), sodium (40 percent, 18 of 45), and manganese (about 2 percent, 1 of 45). Aesthetically based benchmarks were exceeded for dissolved solids (about 29 percent, 13 of 45), chloride (about 4 percent, 2 of 45), iron (about 87 percent, 39 of 45), and manganese (about 29 percent, 13 of 45). CECs were detected at low or estimated concentrations in 8 of the 12 (about 67 percent) monitoring wells analyzed.
In addition to sampling the groundwater monitoring wells, three surface-water-quality monitoring sites also were sampled and analyzed for pharmaceuticals and wastewater indicator compounds to provide a preliminary assessment of the presence of CECs in the surface waters. CECs were detected in all three of the surface-water-quality monitoring samples collected, and wastewater indicator compounds were more prevalent and more frequently detected than pharmaceutical compounds. These results provided a cursory understanding of the presence of CECs in surface waters and do not constitute a robust analysis of sources, seasonality, range of concentrations, persistence, or effects.
The 2020 groundwater-quality results had measurements of field properties and concentrations of major ions, trace metals, and nutrients that were consistent with 2010 results with statistically significant increases for calcium, magnesium, and silica and decreases for aluminum, ammonia, arsenic, barium, bromide, calcium, molybdenum, phosphate, specific conductance, sulfate, and dissolved solids. Increases generally were detected in the intermediate and deep parts of the sand and gravel aquifer, and decreases were detected in the shallow part of the sand and gravel aquifers. The mixed distribution of increases and decreases among the various constituents and aquifer-depth groups could be reflecting dissolution and mobility of some of the redox sensitive constituents and dilution of some constituents in the shallow aquifer depths. These changes may be attributed to a combination of stable population of the past decade (2010–20), to land-use management practices, and to the recent wet years of 2017 through 2019 causing a dilution of the major ions in the shallow parts of the aquifer.
In addition to comparing the 2020 water-quality data to the 2010 data, an analysis for the source of chloride ions in the groundwater was completed by applying the 2020 chloride and bromide concentrations to the chloride-bromide ratio analysis. The results indicate the sources of chloride remained similar to 2010, where many of the chloride concentrations in the groundwater are from road salt applications. A few of the wells indicated some shift from road salt towards sewage or from sewage towards road salt. The change in potential sources may indicate a change in the mixture of sources within that part of the aquifer.
A total of 172 CECs were analyzed for in the groundwater monitoring wells, and only 5 percent of the CECs were detected in low or estimated concentrations, indicating a lack of widespread presence of CECs and supporting the overall good quality of the shallow groundwater resource. CECs were identified in about 66 percent (8 of 12) of the monitoring wells sampled and in all 3 surface-water samples. CECs identified in 10–MAR–S, MARN–09–01, 9–MCH–S, 8–GRN–D, and HEBR–09–03 are consistent with the source water identified in the chloride-bromide ratio analysis. The low level and often estimated concentrations not only indicate the vulnerability of groundwater with respect to sewage and septic effluents but also highlight the potential capacity of the aquifer to degrade or mitigate some the detected CECs. Surface waters indicated the highest concentrations and most frequent detection of CECs. These locations were selected based on proximity to wastewater-treatment plant effluent, and the detections highlight the limits of the treatment systems.
The suitability of the groundwater for drinking water is generally good; however, some naturally occurring minerals, such as arsenic, manganese, and iron, would likely need some filtration or treatment system to reduce the potential toxicity (arsenic) and taste objections (iron and manganese). The suitability of surface water for drinking water is generally good. However, the longer-term effects of chronically low concentrations of CECs are unknown and the sources, range of concentration, and persistence of the CECs would benefit from additional studies.
References Cited
Agency for Toxic Substances and Disease Registry, 2011, Hydrogen sulfide—ToxFAQs™: Agency for Toxic Substances and Disease Registry, 2 p., accessed July 23, 2021, at https://www.atsdr.cdc.gov/toxfaqs/tfacts114.pdf.
Barber, L.B., Keefe, S.H., Brown, G.K., Furlong, E.T., Gray, J.L., Kolpin, D.W., Meyer, M.T., Sandstrom, M.W., and Zaugg, S.D., 2013, Persistence and potential effects of complex organic contaminant mixtures in wastewater-impacted streams: Environmental Science & Technology, v. 47, no. 5, p. 2177–2188, accessed June 3, 2021, at https://doi.org/10.1021/es303720g.
Barr, M.N., and Davis, J.V., 2010, Surface water-quality conditions and long-term trends at selected sites within the ambient water-quality monitoring network in Missouri, water years 1993–2008: U.S. Geological Survey Scientific Investigations Report 2010–5078, 42 p., accessed July 15, 2021, at https://doi.org/10.3133/sir20105078.
Benjamini, Y., and Hochberg, Y., 1995, Controlling the false discovery rate—A practical and powerful approach to multiple testing: Journal of the Royal Statistical Society—Series B (Methodological), v. 57, no. 1, p. 289–300. [Also available at https://doi.org/10.1111/j.2517-6161.1995.tb02031.x.]
Benson, R., Conerly, O.D., Sander, W., Batt, A.L., Boone, J.S., Furlong, E.T., Glassmeyer, S.T., Kolpin, D.W., Mash, H.E., Schenck, K.M., and Simmons, J.E., 2017, Human health screening and public health significance of contaminants of emerging concern detected in public water supplies: Science of the Total Environment, v. 579, p. 1643–1648. [Also available at https://doi.org/10.1016/j.scitotenv.2016.03.146.]
Berg, R.C., Curry, B.B., and Vaiden, R.A., 1997, Geologic mapping for environmental planning, McHenry County, Illinois: Illinois State Geological Survey Circular 559, 79 p. [Also available at https://doi.org/10.13140/RG.2.1.4641.0327.]
Bradley, P.M., Barber, L.B., Kolpin, D.W., McMahon, P.B., and Chapelle, F.H., 2007, Biotransformation of caffeine, cotinine, and nicotine in stream sediments—Implications for use as wastewater indicators: Environmental Toxicology and Chemistry, v. 26, no. 6, p. 1116–1121, accessed July 15, 2021, at https://doi.org/10.1897/06-483R.1.
Centers for Disease Control and Prevention, 2021, Therapeutic drug use (2015–2018): Centers for Disease Control and Prevention web page, accessed July 27, 2021, at https://www.cdc.gov/nchs/fastats/drug-use-therapeutic.htm.
Centers for Disease Control and Prevention, 2022, Heart disease—Sodium: Centers for Disease Control and Prevention web page, accessed March 25, 2022, at https://www.cdc.gov/heartdisease/sodium.htm.
Chemical Book, 2021, Tris(1,3-dochloro-2-propyl)phosphate [Fyrol FR 2]: Chemical Book web page, accessed July 26, 2021, at https://www.chemicalbook.com/ProductChemicalPropertiesCB5194948_EN.htm.
Clark, T.P., and Piskin, R., 1977, Chemical quality and indicator parameters for monitoring landfill leachate in Illinois: Environmental Geology, v. 1, no. 6, p. 329–340. [Also available at https://doi.org/10.1007/BF02380501.]
Davis, S.N., Whittemore, D.O., and Fabryka-Martin, J., 1998, Uses of chloride/bromide ratios in studies of potable water: Ground Water, v. 36, no. 2, p. 338–350. [Also available at https://doi.org/10.1111/j.1745-6584.1998.tb01099.x.]
Domagalski, J.L., and Johnson, H., 2012, Phosphorus and groundwater—Establishing links between agricultural use and transport to streams: U.S. Geological Survey Fact Sheet 2012–3004, 4 p. [Also available at https://doi.org/10.3133/fs20123004.]
Drugs.com, 2021, Drugs.com—Know more. Be sure.: Drugs.com web page, accessed July 26, 2021, at https://www.drugs.com/.
Dubrovsky, N.M., Burow, K.R., Clark, G.M., Gronberg, J.M., Hamilton, P.A., Hitt, K.J., Mueller, D.K., Munn, M.D., Nolan, B.T., Puckett, L.J., Rupert, M.G., Short, T.M., Spahr, N.E., Sprague, L.A., and Wilber, W.G., 2010, The quality of our Nation’s waters—Nutrients in the Nation’s streams and groundwater, 1992–2004: U.S. Geological Survey Circular 1350, 174 p. [Also available at https://doi.org/10.3133/cir1350.]
Fishman, M.J., ed., 1993, Methods of analysis by the U.S. Geological Survey National Water Quality Laboratory—Determination of inorganic and organic constituents in water and fluvial sediments: U.S. Geological Survey Open-File Report 93–125, 217 p. [Also available at https://doi.org/10.3133/ofr93125.]
Fishman, M.J., and Friedman, L.C., 1989, Methods for determination of inorganic substances in water and fluvial sediments: U.S. Geological Survey Techniques of Water-Resources Investigations, book 5, chap. A1, 545 p. [Also available at https://doi.org/10.3133/twri05A1.]
Furlong, E.T., Werner, S.L., Anderson, B.D., Cahill, J.D., 2008, Determination of human-health pharmaceuticals in filtered water by chemically modified styrene-divinylbenzene resin-based solid-phase extraction and high-performance liquid chromatography/mass spectrometry, U.S. Geological Survey Techniques and Methods, book 5, sec. B, chap. B5, 56 p. 13.16. [Also available at https://pubs.usgs.gov/tm/tm5b5/pdf/tm-5-b5_508.pdf.]
Furlong, E.T., Noriega, M.C., Kanagy, C.J., Kanagy, L.K., Coffey, L.J., and Burkhardt, M.R., 2014, Determination of human-use pharmaceuticals in filtered water by direct aqueous injection—High-performance liquid chromatography/tandem mass spectrometry: U.S. Geological Survey Techniques and Methods, book 5, chap. B10, 49 p. [Also available at https://doi.org/10.3133/tm5B10.]
Gahala, A.M., 2017, Hydrogeology and water quality of sand and gravel aquifers in McHenry County, Illinois, 2009–14, and comparison to conditions in 1979 (ver. 1.1, August 2022): U.S. Geological Survey Scientific Investigations Report 2017–5112, 91 p., accessed August 26, 2022, at https://doi.org/10.3133/sir20175112.
Gahala, A.M., and Gruhn, L.R., 2022, Quality-assurance and quality-control data for discrete water-quality samples collected in McHenry County, Illinois, 2020: U.S. Geological Survey data release, https://doi.org/10.5066/P9RBXV53.
Garbarino, J.R., Kanagy, L.K., and Cree, M.E., 2006, Determination of elements in natural-water, biota, sediment, and soil samples using collision/reaction cell inductively coupled plasma-mass spectrometry: U.S. Geological Survey Techniques and Methods, book 5, chap. B1, 88 p., accessed June 13, 2022, at https://doi.org/10.3133/tm5B1.
Groschen, G.E., Arnold, T.L., Morrow, W.S., and Warner, K.L., 2008, Occurrence and distribution of iron, manganese, and selected trace elements in ground water in the glacial aquifer system of the Northern United States: U.S. Geological Survey Scientific Investigations Report 2009–5006, 89 p. [Also available at https://doi.org/10.3133/sir20095006.]
Hatfield, J.L., and Prueger, J.H., 2004, Impacts of changing precipitation patterns on water quality: Journal of Soil and Water Conservation, v. 59, no. 1, p. 51–58, accessed February 15, 2022, at https://www.jswconline.org/content/59/1/51.
Hem, J.D., 1985, Study and interpretation of the chemical characteristics of natural water (3d ed.): U.S. Geological Survey Water-Supply Paper 2254, 263 p., accessed July 23, 2021, at https://doi.org/10.3133/wsp2254.
Illinois State Water Survey, 2022, McHenry County, Illinois, watersheds: Champaign, accessed June 13, 2022 at https://www.mchenrycountyil.gov/home/showdocument?id=8762.
Jha, S.K., Mishra, V.K., Sharma, D.K., and Damodaran, T., 2011, Fluoride in the environment and its metabolism in humans: Reviews of Environmental Contamination and Toxicology, v. 211, p. 121–142. [Also available at https://doi.org/10.1007/978-1-4419-8011-3_4.]
Jha, S.K., Singh, R.K., Damodaran, T., Mishra, V.K., Sharma, D.K., and Rai, D., 2013, Fluoride in groundwater—Toxicological exposure and remedies: Journal of Toxicology and Environmental Health—Part B, Critical Reviews, v. 16, no. 1, p. 52–66. [Also available at https://doi.org/10.1080/10937404.2013.769420.]
Julian, P., and Helsel, D., 2021, R—A language and environment for statistical computing, version 1.0.1 (NADA2—Data Analysis for Censored Environmental Data): R Foundation for Statistical Computing software release, accessed April 11, 2022, at https://cran.r-project.org/web/packages/NADA2/index.html.
Kolpin, D.W., Furlong, E.T., Meyer, M.T., Thurman, E.M., Zaugg, S.D., Barber, L.B., and Buxton, H.T., 2002, Pharmaceuticals, hormones, and other organic wastewater contaminants in U.S. streams, 1999–2000—A national reconnaissance: Environmental Science & Technology, v. 36, no. 6, p. 1202–1211, accessed September 10, 2018, at https://doi.org/10.1021/es011055j.
Lin, Z., and Puls, R.W., 2000, Adsorption, desorption and oxidation of arsenic affected by clay minerals and aging process: Environmental Geology, v. 39, no. 7, p. 753–759. [Also available at https://doi.org/10.1007/s002540050490.]
Lopaka, L., and Helsel, D., 2020, R—A language and environment for statistical computing, version 1.6–1.1 (NADA—Nondetects and Data Analysis for Environmental Data): R Foundation for Statistical Computing software release, accessed April 10, 2022, at https://cran.r-project.org/web/packages/NADA/index.html.
Martin, C.B., Hales, C.M., Gu, Q., and Ogden, C.L., 2019, Prescription drug use in the United States, 2015–2016: National Center for Health Statistics, Centers for Disease Control and Prevention web page, accessed July 27, 2021, at https://www.cdc.gov/nchs/products/databriefs/db334.htm.
McCallum, J.E., Ryan, M.C., Mayer, B., and Rodvang, S.J., 2008, Mixing-induced groundwater denitrification beneath a manured field in southern Alberta, Canada: Applied Geochemistry, v. 23, no. 8, p. 2146–2155, accessed April 2020 at https://doi.org/10.1016/j.apgeochem.2008.03.018.
McHenry County Planning and Development, 2020, McHenry County Water Resources Action Plan (WRAP) 2020 update: McHenry County Planning and Development, prepared by Water Resources Action Plan Task Force and Department of Planning and Development, 30 p., accessed June 13, 2022, at https://www.mchenrycountyil.gov/county-government/departments-j-z/planning-development/water-resources/water-resources-action-plan.
Mullaney, J.R., Lorenz, D.L., and Arntson, A.D., 2009, Chloride in groundwater and surface water in areas underlain by the glacial aquifer system, northern United States: U.S. Geological Survey Scientific Investigations Report 2009–5086, 41 p., accessed July 15, 2021, at https://doi.org/10.3133/sir20095086.
Nádaská, G., Lesný, J., and Michalík, I., 2010, Environmental aspect of manganese chemistry: Trnava, Slovakia, HEJ, University of SS Cyril and Methodius, Department of Biotechnology, ENV–100702–A, 16 p., accessed July 15, 2021, at http://heja.szif.hu/ENV/ENV-100702-A/env100702a.pdf.
National Centers for Environmental Information, 2022a, Global Historical Climatology Network–Daily, version 3 [Woodstock 5NW, IL, 2010–20, daily precipitation data]: National Centers for Environmental Information digital data, accessed February 28, 2022, at https://www.ncei.noaa.gov/access/search/data-search/daily-summaries?dataTypes=PRCP&startDate=2010-01-01T00:00:00&endDate=2020-12-31T23:59:59&bbox=42.5 37,-88.665,42.111,-88.239.
National Centers for Environmental Information, 2022b, U.S. Climate Normals Quick Access [Woodstock 5NW, IL, 1981–2010, annual/seasonal]: National Centers for Environmental Information digital data, accessed February 28, 2022, at https://www.ncei.noaa.gov/access/us-climate-normals/#dataset=normals-annualseasonal&timeframe=81&location=IL&station=USC00119480.
National Centers for Environmental Information, 2022c, U.S. Climate Normals Quick Access [Woodstock 5NW, IL, 1991–2010, annual/seasonal]: National Centers for Environmental Information digital data, accessed February 28, 2022, at https://www.ncei.noaa.gov/access/us-climate-normals/#dataset=normals-annualseasonal&timeframe=30&location=IL&station=USC00119480.
National Centers for Environmental Information, 2022d, U.S. Climate Normals Quick Access [Woodstock 5NW, IL, 2006–2020, annual/seasonal]: National Centers for Environmental Information digital data, accessed February 28, 2022, at https://www.ncei.noaa.gov/access/us-climate-normals/#dataset=normals-annualseasonal&timeframe=15&location=IL&station=USC00119480.
National Ground Water Association, 2010, Dissolved mineral sources and significance: National Ground Water Association web page, accessed June 13, 2022, at https://www.ngwa.org/what-is-groundwater/About-groundwater/dissolved-mineral-sources-and-significance.
Nicholas, J.R., and Krohelski, J.T., 1984, Water in sand and gravel deposits in McHenry County, Illinois: U.S. Geological Survey Water-Resources Investigations Report 83–4048, 34 p., accessed June 13, 2022, at https://doi.org/10.3133/wri834048.
Oblinger Childress, C.J., Foreman, W.T., Connor, B.F., and Maloney, T.J., 1999, New reporting procedures based on long-term method detection levels and some considerations for interpretations of water-quality data provided by the U.S. Geological Survey National Water Quality Laboratory: U.S. Geological Survey Open-File Report 99–193, 19 p., accessed July 15, 2021, at https://doi.org/10.3133/ofr99193.
Panno, S.V., Hackley, K.C., Hwang, H.H., Greenberg, S.E., Krapac, I.G., Landsberger, S., and O’Kelly, D.J., 2006, Characterization and identification of Na-Cl sources in ground water: Ground Water, v. 44, no. 2, p. 176–187. [Also available at https://doi.org/10.1111/j.1745-6584.2005.00127.x.]
Pieper, K.J., Tang, M., Jones, C.N., Weiss, S., Greene, A., Mohsin, H., Parks, J., and Edwards, M.A., 2018, Impact of road salt on drinking water quality and infrastructure corrosion in private wells: Environmental Science & Technology, v. 52, no. 24, p. 14078–14087, accessed July 23, 2021, at https://doi.org/10.1021/acs.est.8b04709.
Piper, A.M., 1944, A graphic procedure in the geochemical interpretation of water-analyses: EOS Transactions of the American Geophysical Union, v. 25, no. 6, p. 914–928. [Also available at https://doi.org/10.1029/TR025i006p00914.]
PubChem, 2021a, Indole (compound): PubChem web page, accessed July 26, 2021, at https://pubchem.ncbi.nlm.nih.gov/compound/798#section=Uses.
PubChem, 2021b, 2,6-Dimethylnaphthalene (compound): PubChem web page, accessed July 26, 2021, at https://pubchem.ncbi.nlm.nih.gov/compound/2_6-Dimethylnaphthalene#section=Use-and-Manufacturing.
PubChem, 2021c, beta-Sitosterol (compound): PubChem web page, accessed July 26, 2021, at https://pubchem.ncbi.nlm.nih.gov/compound/222284#section=Uses.
PubChem, 2021d, Diethyl phthalate (compound): PubChem web page, accessed July 26, 2021, at https://pubchem.ncbi.nlm.nih.gov/compound/6781#section=Use-and-Manufacturing.
R Core Team, 2019, R—A language and environment for statistical computing: Vienna, Austria, R Foundation for Statistical Computing, accessed April 4, 2022, at https://www.R-project.org/ and https://cran.r-project.org/src/base/R-3/.
R Core Team, 2021, R—A language and environment for statistical computing, version 2021.09.1 (Wilcoxon Rank Sum and Signed Rank Tests): R Foundation for Statistical Computing software release, accessed April 4, 2022, at https://search.r-project.org/R/refmans/stats/html/wilcox.test.html.
Renard, F., Putnis, C.V., Montes-Hernandez, G., Ruiz-Agudo, E., Hovelmann, J., and Sarret, G., 2015, Interactions of arsenic with calcite surfaces revealed by in situ nanoscale imaging: Geochimica et Cosmochimica Acta, v. 159, p. 61–79. [Also available at https://doi.org/10.1016/j.gca.2015.03.025.]
Salameh, E., Tarawneh, A., and Al-Raggad, M., 2016, Origin of high bromide concentration in the water sources in Jordan and in the Dead Sea water: Arabian Journal of Geosciences, v. 9, no. 5, art. 414, 20 p., accessed November 10, 2021, at https://doi.org/10.1007/s12517-016-2431-9.
Shi, X., Fay, L., Yang, Z., Nguyen, T.A., and Liu, Y., 2009, Corrosion of deicers to metals in transportation infrastructure—Introduction and recent developments: Journal of Corrosion Reviews, v. 7, no. 1–2, p. 23–52. [Also available at https://doi.org/10.1515/CORRREV.2009.27.1-2.23.]
Stiff, B.J., and Hansel, A.K., 2004, Quaternary glaciations in Illinois, in Ehlers, J., and Gibbard, P.L., eds., Quaternary glaciations—Extent and chronology—Part II—North America: Amsterdam, Elsevier, p. 71–82. [Also available at https://doi.org/10.1016/S1571-0866(04)80188-X.]
Thomas, M.A., and Eckberg, M., 2015, The effectiveness of water-treatment systems for arsenic used in 11 homes in Southwestern and Central Ohio, 2013: U.S. Geological Survey Scientific Investigations Report 2015–5156, 26 p., accessed August 2016 at https://doi.org/10.3133/sir20155156.
Tomasek, A.A., Lee, K.E., and Hansen, D.S., 2012, Wastewater indicator compounds in wastewater effluent, surface water, and bed sediment in the St. Croix National Scenic Riverway and implications for water resources and aquatic biota, Minnesota and Wisconsin, 2007–08: U.S. Geological Survey Scientific Investigations Report 2011–5208, 34 p., accessed November 10, 2021, at https://doi.org/10.3133/sir20115208.
U.S. Census Bureau, 2021, Total population in McHenry County, Illinois [2010 and 2020 table results]: U.S. Census Bureau digital data, accessed October 12, 2021, at https://data.census.gov/cedsci/all?q=McHenry%20County,%20Illinois.
U.S. Environmental Protection Agency, 1990, National Pesticide Survey—Prometon: U.S. Environmental Protection Agency, 4 p., accessed July 22, 2021, at https://nepis.epa.gov/Exe/ZyPDF.cgi/91014VLQ.PDF?Dockey=91014VLQ.PDF.
U.S. Environmental Protection Agency, 1995, Metolachlor, R.E.D. Facts, Prevention, Pesticides, and Toxic Substances, accessed August 24, 2022 at https://www3.epa.gov/pesticides/chem_search/reg_actions/reregistration/fs_PC-108801_1-Apr-95.pdf.
U.S. Environmental Protection Agency, 2002, Onsite wastewater treatment systems manual: U.S. Environmental Protection Agency, Office of Water, Office of Research and Development, 367 p., accessed July 8, 2021, at https://www.epa.gov/sites/production/files/2015-06/documents/2004_07_07_septics_septic_2002_osdm_all.pdf.
U.S. Environmental Protection Agency, 2009a, National primary drinking water regulations: Washington, D.C., U.S. Environmental Protection Agency, EPA 816–F–09–004, 7 p., accessed May 10, 2021, at https://www.epa.gov/sites/default/files/2016-06/documents/npwdr_complete_table.pdf.
U.S. Environmental Protection Agency, 2009b, Occurrence of contaminants of emerging concern in wastewater from nine publicly owned treatment works: Washington, D.C., U.S. Environmental Protection Agency, Office of Water, EPA–821–R–09–009, 85 p., accessed July 8, 2021, at https://www.epa.gov/sites/default/files/2018-11/documents/occurrence-cec-wastewater-9-treatment-work.pdf#:~:text=In%202005%2C%20EPA%20began%20studying %20environmental%20contamination%20by,believed%20to%20enter%20municipal%20wastewater%20through%20bathing%2C%20cleaning%2C.
U.S. Environmental Protection Agency, 2018, 2018 edition of the Drinking Water Standards and Health Advisories tables: Washington, D.C., U.S. Environmental Protection Agency, Office of Water, EPA 822–F–18–001, 12 p., accessed March, 25, 2022, at https://www.epa.gov/system/files/documents/2022-01/dwtable2018.pdf.
U.S. Environmental Protection Agency, 2021a, Atrazine: U.S. Environmental Protection Agency web page, accessed July 22, 2021, at https://www.epa.gov/ingredients-used-pesticide-products/atrazine.
U.S. Environmental Protection Agency, 2021b, National recommended water-quality criteria—Aquatic life criteria table: U.S. Environmental Protection Agency web page, accessed October 12, 2021, at https://www.epa.gov/wqc/national-recommended-water-quality-criteria-aquatic-life-criteria-table.
U.S. Environmental Protection Agency, 2022a, CADDIS volume 2—Metals: U.S. Environmental Protection Agency web page, accessed March 25, 2022, at https://www.epa.gov/caddis-vol2/metals.
U.S. Environmental Protection Agency, 2022b, Research on the reproductive effects of endocrine disrupting chemicals, accessed Aug. 24, 2022 at https://www.epa.gov/chemical-research/research-reproductive-effects-endocrine-disrupting-chemicals.
U.S. Geological Survey, 1999, The quality of our Nation’s waters—Nutrients and pesticides: U.S. Geological Survey Circular 1225, 82 p. [Also available at https://doi.org/10.3133/cir1225.]
U.S. Geological Survey, 2018, Pharmaceuticals in water: U.S. Geological Survey web page, accessed January 2022 at https://www.usgs.gov/special-topics/water-science-school/science/pharmaceuticals-water.
U.S. Geological Survey, 2019, Metals and other trace elements: U.S. Geological Survey web page, accessed July 22, 2021, at https://www.usgs.gov/mission-areas/water-resources/science/metals-and-other-trace-elements?qt-science_center_objects=0#qt-science_center_objects.
U.S. Geological Survey, 2020a, Estimated use of water in the United States—County-level data for 2015: U.S. Geological Survey web page, accessed December 2020 at https://water.usgs.gov/watuse/data/data2015.html.
U.S Geological Survey, 2020b, USGS water data for the Nation: U.S. Geological Survey National Water Information System database, accessed May 11, 2021, at https://doi.org/10.5066/F7P55KJN.
U.S. Geological Survey, 2021a, Pesticides: U.S. Geological Survey web page, accessed July 22, 2021, at https://www.usgs.gov/centers/oki-water/science/pesticides?qt-science_center_objects=0#qt-science_center_objects.
U.S. Geological Survey, 2021b, Wastewater indicators: U.S. Geological Survey web page, accessed July 22, 2021, at https://www.usgs.gov/labs/nwql/science-topics/wastewater-indicators.
U.S. Geological Survey, 2022, Emerging contaminants: U.S. Geological Survey web page, accessed February 23, 2022, at https://www.usgs.gov/mission-areas/water-resources/science/emerging-contaminants.
U.S. Geological Survey, [variously dated], National field manual for the collection of water-quality data: U.S. Geological Survey Techniques of Water-Resources Investigations, book 9, chaps. A1–A10, [variously paged]. [Also available at https://doi.org/10.3133/twri09.]
WebMD, 2021, Atrazine tablet—Uses, side effects, and more: WebMD web page, accessed July 26, 2021, at https://www.webmd.com/drugs/2/drug-57038/atrazine-oral/details#:~:text=It%20is%20an%20antihistamine%20and,relaxed%20before%20and%20after%20surgery.
Williams, T., Formean, W.T., Decess, J., Reed-Parker, C., and Stevenson, D.L., 2015, Changes to National Water Quality Laboratory (NWQL) procedures used to establish and verify laboratory detection and reporting limits: U.S. Geological Survey, National Water Quality Laboratory Technical Memorandum 15.02, 21 p., accessed July12, 2021, at https://nwql.usgs.gov/tech_memos/nwql.2015-02.pdf.
Wilson, V.S., Evans, N., Schenck, K.M., Mash, H., Rosenblum, L., and Glassmeyer, S., 2011, USEPA/USGS study of CECs in source water and treated drinking water—Assessment of estrogenic activity using an in vitro bioassay, T47D-KBluc: Presented at Society of Toxicology and Chemistry (SETAC) 23rd Annual Meeting, Boston, Mass., November 13–17, 2011, accessed August 24, 2022, at https://cfpub.epa.gov/si/si_public_record_report.cfm?Lab=NHEERL&dirEntryId=235705.
Woller, D.M., and Sanderson, E.W., 1976, Public groundwater supplies in McHenry County, Illinois: Urbana, Ill., Illinois State Water Survey Bulletin 60–19, 52 p. [Also available at https://www.mchenrycountyil.gov/home/showpublisheddocument/43276/635664177521530000.]
World Health Organization, 2011, Manganese in drinking-water—Background document for development of WHO guidelines for drinking-water quality: World Health Organization, WHO/SDE/WSH/03.04/104/Rev/1, 29 p., accessed June 13, 2022, at https://www.who.int/publications/i/item/WHO-HEP-ECH-WSH-2021.5.
Zaugg, S.D., Smith, S.G., and Schroeder, M.P., 2006, Determination of wastewater compounds in whole water by continuous liquid-liquid extraction and capillary-column gas chromatography/mass spectrometry (ver. 1.1, October 2007): U.S. Geological Survey Techniques and Methods, book 5, chap. B4, 30 p. [Also available at https://doi.org/10.3133/tm5B4.]
Appendix 1. Quality Assurance and Quality Control of Water-Quality Results
Quality Assurance and Quality Control for Major Ions, Nutrients, Metals, and Trace Elements
Quality assurance and quality control (QAQC) of the water-quality results were completed by collecting blank (field and equipment) and replicate (duplication of the environmental sample for analysis of variability) samples about once per week during the sampling period between June and July of 2020 to evaluate variability and control bias in analytical results (table 1.1; Gahala and Gruhn, 2022). One equipment blank was collected for each sampling pump type before any field sampling. A total of four field equipment blanks were collected following the decontamination procedures, three for the analysis of nutrients, major ions, and metals and one for the analysis of pharmaceuticals and wastewater indicator compounds (WICs). A total of five replicate samples were collected to verify the laboratory results: two for nutrients, major ions, and metals/trace elements; one for pharmaceuticals and WICs; one for pharmaceuticals; and one for WICs.
Table 1.1.
Chemical characteristics of selected quality-control samples collected in McHenry County, Illinois, June and July 2020.[mg/L, milligram per liter; N, nitrogen; <, less than reporting level; NR, not reported; P, phosphorous; µg/L, microgram per liter; 180C, laboratory dissolved solids dried at 180 degrees Celsius; NA, not available]
Pre-field equipment blanks were collected before any field use or mobilization. Pumps and associated tubing were washed with liquid Alconox solution, rinsed with tap water, and rinsed a third time with de-ionized water. Inorganic blank water was then passed through the pumps and tubing and the filter, and blank samples were collected in their respective prelabeled sample bottles, preserved with nitric acid, and placed in an ice-bath cooler and sent overnight to the U.S. Geological Survey National Water Quality Laboratory in Denver, Colorado, for analysis. Field blanks were collected to test for the quality of decontamination procedures. Field blank samples were collected in a similar manner after the collection of the environmental and replicate samples, following the decontamination procedures and addition of a new filter for filtered samples.
The pre-field equipment blank from the Fultz pump detected low concentrations of several constituents. Cobalt, copper, lead, nickel, zinc, antimony, and bromide were detected at low concentrations but were within the range of concentrations detected in the environmental samples. Calcium, fluoride, silica, barium, and strontium also had some low-level detections but were below the range of concentrations detected in the environmental samples. Subsequent field rinse equipment blanks from the Fultz pump did not have detections greater than the reporting level for lead, and antimony, and therefore, the environmental sample results are considered acceptable. One field-rinse equipment blank sample (7–HRT–D) detected bromide just above the reporting limits (less than [<] 0.010 and <0.020) of 0.011 mg/L and is within the range of some of the environmental samples. This detection could be attributed to the fluctuating reporting limit for many of the results and may increase the uncertainty of concentrations less than 0.020 mg/L. It is possible that the initial pre-field equipment blank had remnant tap water and (or) de-ionized water inadvertently collected in the samples. Equipment blanks from the Grundfos pump detected many of the same constituents as detected in the Fultz pump, and therefore, detections of some of these constituents with similar low concentrations in the environmental samples for 17–ALG–D and WAUC–02–12 collected with the Grundfos are estimated. These detections within the blanks, regardless of the pump, indicate the source is potentially from tubing or laboratory interference or tap/de-ionized rinse water. Of the three field blanks, low-level detections were noted for several constituents, including many of the same constituents detected in the blank samples. Except for copper, cobalt, and zinc, no other detections were within the range of the concentrations detected in the environmental samples. Results with detections in the pre-field equipment blanks and field blanks are estimated. Statistical analyses for cobalt, copper, nickel, and zinc were not evaluated (table 1.1).
Replicate samples were collected to assess the reproducibility of the sample-collection procedures and laboratory analysis to provide a measure of variability. Replicate samples were collected immediately after the environmental sample. Of the 34 comparisons between major ions, trace metals, and nutrients analyzed, 4 constituents (orthophosphate, cobalt, copper, and bromide) had differences greater than 10 percent. Cobalt and copper were routinely detected in blank samples and are excluded from further analysis or considerations. The differences in orthophosphate greater than 10 percent were because of low concentrations near the method detection level, but the environmental results are reliable enough to determine water types and would not alter the determination of whether a constituent exceeded water-quality standards.
Bromide had larger variability in detected concentration results (23- and 37-percent difference) between replicates and environmental samples. The replicate value for bromide in 9-MCH–S was 0.036 microgram per liter (µg/L), and the replicate chloride concentration was 123 milligrams per liter (mg/L). The environmental sample for 9–MCH–S had a detected bromide concentration of 0.057 µg/L and the chloride concentration of 121 mg/L was consistent with the replicate concentration. The differences in detection of bromide concentration affected where the environmental and replicate results plotted on the chloride-bromide source curves. The 9–MCH–S environmental sample chloride-bromide ratio plotted ambiguously between the “sewage and road salt high curve” and “dilute groundwater and softener” curves. Previous samples from 2010 through 2015 showed 9–MCH–S chloride-bromide ratios plotting near and along the “sewage and road salt, high” curve (Gahala, 2017). The 2020 environmental and replicate chloride-bromide ratio results shifted the datapoints away from the “sewage and road salt, high” curve towards and near the “dilute groundwater and softener” curve. The environmental sample for 7–HRT–D plotted near the dilute groundwater and sewage (low) curve, whereas the replicate was slightly farther away from that curve and slightly closer to the 2010 data point where it was between the curves of dilute groundwater and sewage (low) and the curve for brine within the dilute range. The shift was not substantially different enough to change the interpretation of the source.
Quality Assurance and Quality Control for Contaminants of Emerging Concern
For the contaminants of emerging concern (CECs), five QAQC samples were collected (one field blank, three replicates [one full, one for only pharmaceuticals, and one for only WICs], and one equipment blank). The Fultz pump was the only pump used for the collection of samples for the analysis of organic compounds (pharmaceuticals and WICs). After the equipment decontamination steps of wash, tap water rinse, and de-ionized water rinse, laboratory grade methanol was run through the Fultz pump and tubing and followed by certified organic blank water. After the methanol wash passed through the tubing, the certified organic blank water was then collected in the appropriate sample containers for the analysis of pharmaceuticals and WICs. Samples collected for pharmaceutical analysis were filtered with a 25-millimeter disposable filter. Field blank samples were collected in a similar manner after the collection of the environmental and replicate samples, following the decontamination procedures, and a new 25-millimeter filter was used. Samples were placed in an ice-bath cooler and sent overnight to the National Water Quality Laboratory for analysis. All detections, reported and estimated, are presented in table 1.2.
Table 1.2.
Detections of contaminants of emerging concern in quality assurance and quality control samples.[CEC, contaminant of emerging concern; pharm, pharmaceuticals; WIC, wastewater indicator compound; OOT, organics other; ng/L, nanogram per liter; E, estimated; <, less than reporting level; —, no data; μg/L, microgram per liter; OPE, organics pesticides]
Two replicate samples were collected for the analysis of pharmaceuticals and WICs. Of the 155 comparisons for pharmaceuticals and 74 comparisons for WICs, 8 differences were detected. Two constituents, prometon and nicotine, had detections in the environmental sample and the replicate and no detections in the equipment blank or field blank. Other constituents (atrazine, less than 0.16 nanogram per liter [ng/L]; carbamazepine, 2.9 ng/L) had detections in either the sample or the replicate, but not both; and the remaining constituents had detections in either the equipment blank or field blank. Based on these results, prometon, nicotine, atrazine, and carbamazepine are considered as likely detections in the groundwater monitoring well samples.
Results from groundwater wells were variable, and some detections in the QAQC samples were not detected in the primary environmental samples. This variability was represented in the results of the analysis of the environmental sample and the associated replicate QAQC samples where a detection in one sample was not detected in the other in every instance. In other words, a detection occurred in either the environmental sample or the replicate sample, but not in both. This pattern was detected in 9–MCH–S sample results for carbamazepine and atrazine. Other types of QAQC samples (equipment and field blanks) also unexpectedly resulted in detections of several CECs. This pattern could potentially be caused by ineffective de-ionization of water at the local laboratory where equipment for sampling is cleaned and stored. If water was not being completely de-ionized, some of these CECs were potentially being detected in tap water that is not fully processed through de-ionization. Nicotine was detected in six monitoring wells, including the three hypothesized background monitoring wells. Detections were less than the reporting level of 80 ng/L and ranged from 23.2 to 38.6 ng/L. Replicate results for nicotine were less than 10-percent different (6 percent). N,N-Diethyl-m-toluamide (DEET) also was detected in every environmental sample and every QAQC sample despite personnel adhering to field sampling protocol of avoiding the use of any repellent (or pharmaceutical and personal care products). The cross contamination is suspected to be from the laboratory machines and the ubiquitous and persistent nature of DEET. The results for DEET are therefore not considered in the tally of detections or any further discussion.
References Cited
Gahala, A.M., 2017, Hydrogeology and water quality of sand and gravel aquifers in McHenry County, Illinois, 2009–14, and comparison to conditions in 1979 (ver. 1.1, August 2022): U.S. Geological Survey Scientific Investigations Report 2017–5112, 91 p., accessed August 26, 2022, at https://doi.org/10.3133/sir20175112.
Gahala, A.M., and Gruhn, L.R., 2022, Quality-assurance and quality-control data for discrete water-quality samples collected in McHenry County, Illinois, 2020: U.S. Geological Survey data release, https://doi.org/10.5066/P9RBXV53.
Conversion Factors
Datum
Vertical coordinate information is referenced to the North American Vertical Datum of 1988 (NAVD 88).
Horizontal coordinate information is referenced to the North American Datum of 1983 (NAD 83).
Elevation, as used in this report, refers to distance above the vertical datum.
Supplemental Information
Specific conductance is given in microsiemens per centimeter at 25 degrees Celsius (µS/cm at 25 °C).
Concentrations of chemical constituents in water are given in either milligrams per liter (mg/L), micrograms per liter (µg/L), or nanograms per liter (ng/L).
Turbidity is given in nephelometric turbidity units (NTU).
Abbreviations
CEC
contaminant of emerging concern
DEET
N,N-Diethyl-m-toluamide
DWA
drinking water advisory
EPA
U.S. Environmental Protection Agency
MCGMN
McHenry County Groundwater Monitoring Network
MCL
maximum contaminant level
NAWQA
National Water-Quality Assessment
NWQL
National Water Quality Laboratory
p
probability
PPW
paired Prentice-Wilcoxon [test]
QAQC
quality assurance and quality control
SMCL
secondary maximum contaminant level
USGS
U.S. Geological Survey
WIC
wastewater indicator compound
WSR
Wilcoxon signed rank [test]
<
less than
For more information about this publication, contact:
Director, USGS Central Midwest Water Science Center
405 North Goodwin
Urbana, IL 61801
217–328–8747
For additional information, visit: https://www.usgs.gov/centers/cm-water
Publishing support provided by the
Rolla Publishing Service Center
Disclaimers
Any use of trade, firm, or product names is for descriptive purposes only and does not imply endorsement by the U.S. Government.
Although this information product, for the most part, is in the public domain, it also may contain copyrighted materials as noted in the text. Permission to reproduce copyrighted items must be secured from the copyright owner.
Suggested Citation
Gahala, A.M., Gruhn, L.R., Murphy, J.C., and Matson, L.A., 2022, Water quality of sand and gravel aquifers in McHenry County, Illinois, 2020 and comparisons to conditions in 2010: U.S. Geological Survey Scientific Investigations Report 2022–5110, 53 p., https://doi.org/10.3133/sir20225110.
ISSN: 2328-0328 (online)
Study Area
Publication type | Report |
---|---|
Publication Subtype | USGS Numbered Series |
Title | Water quality of sand and gravel aquifers in McHenry County, Illinois, 2020 and comparisons to conditions in 2010 |
Series title | Scientific Investigations Report |
Series number | 2022-5110 |
DOI | 10.3133/sir20225110 |
Year Published | 2022 |
Language | English |
Publisher | U.S. Geological Survey |
Publisher location | Reston, VA |
Contributing office(s) | Central Midwest Water Science Center |
Description | Report: viii, 53 p.; Data Release; Dataset |
Country | United States |
State | Illinois |
County | McHenry County |
Online Only (Y/N) | Y |
Google Analytic Metrics | Metrics page |