Iodine-129 in the Eastern Snake River Plain Aquifer at and near the Idaho National Laboratory, Idaho, 2021–22
Links
- Document: Report (2.6 MB pdf) , HTML , XML
- Data Release: USGS data release - Datasets for the U.S. Geological Survey—Idaho National Laboratory groundwater and surface-water monitoring networks, v1.1
- Download citation as: RIS | Dublin Core
Acknowledgments
The authors are grateful to the Purdue Rare Isotope Measurement Laboratory (PRIME) for analyzing samples and reporting data. The authors gratefully acknowledge efforts made by the U.S. Geological Survey Idaho National Laboratory project office field team to collect samples.
Abstract
Between the 1950s and 1980s, wastewater generated at the Idaho National Laboratory contained Iodine-129 (129I); this wastewater was discharged directly into the eastern Snake River Plain (ESRP) aquifer through a deep disposal well, unlined infiltration ponds, or leaked from distribution systems below industrial facilities. During 2021–22, the U.S. Geological Survey, in cooperation with the U.S. Department of Energy and the Idaho Department of Environmental Quality Idaho Laboratory Oversight Program, collected groundwater samples from 64 monitoring wells in the ESRP aquifer, 6 of which are part of a multilevel monitoring system, to determine the concentration of 129I in the groundwater. These samples were analyzed by accelerator mass spectrometry as part of a long-term ongoing study to track trends and occurrences of this carcinogenic, long-lived radionuclide in the environment. Concentrations ranged from slightly above the locally determined background concentration of 5.4×10−6 picocuries per liter, to just below the U.S. Environmental Protection Agency’s maximum contaminant level of 1 picocurie per liter. Discharge of wastewater containing 129I has been discontinued to the aquifer, and long-term trends from a subset (n=15) of sampled wells show decreasing 129I concentrations over the last three decades. Concentrations of 129I in groundwater from monitoring wells near facilities at the Idaho National Laboratory are affected by episodic recharge from an ephemeral surface-water source and by the fracture-flow dominated hydrologic regime in the ESRP aquifer. The spatially focused sampling effort has also identified a low-level 129I plume that affects long-term water quality near and downgradient from the Advanced Test Reactor Complex in the southwestern part of the facility that had not been clearly defined in previous sampling efforts, although the definition of the plume is somewhat limited by available data.
Introduction
The Idaho National Laboratory (INL), encompassing about 890 square miles of the eastern Snake River Plain (ESRP) in southeastern Idaho (fig. 1), is operated by the U.S. Department of Energy (DOE). The INL was established in 1949 to develop atomic energy, conduct nuclear safety research, and carry out defense program research. Presently, the INL is developing advanced energy concepts and novel energy solutions to ensure the future security of the Nation’s energy resources and technologies. As part of nuclear research at the INL, the Idaho Nuclear Technology and Engineering Center (INTEC, fig. 1) recovered uranium from spent nuclear fuel elements from government-owned reactors until the early 1990s. As part of the fuel reprocessing activities at the INL, fission products, including Iodine-129 (129I), which is produced by the fission of uranium-235 and plutonium-239, were released as a reprocessing by-product (Mann and Beasley, 1994b).
129I-bearing wastewater generated at the INL between the 1950s and 1990s was mostly discharged to the ESRP aquifer at or near the INTEC facility (Mann and Beasley, 1994b). This wastewater was discharged to the aquifer either through a 598-foot (ft) deep disposal well (before 1984; CPP 3, fig. 3); via unlined infiltration ponds (beginning in 1984); or leaked from distribution systems below the INTEC. Records from the late 1970s suggest 129I was also discharged into the unlined radioactive waste ponds at the Advanced Test Reactor Complex (ATRC; figs. 2–3) but published annual concentrations of 129I in the wastewater were generally much less than 1 picocurie per liter (pCi/L); for example, in 1978, Batchelder (1979) showed an average annual concentration of 1.3×10−5 pCi/L of 129I in wastewater.
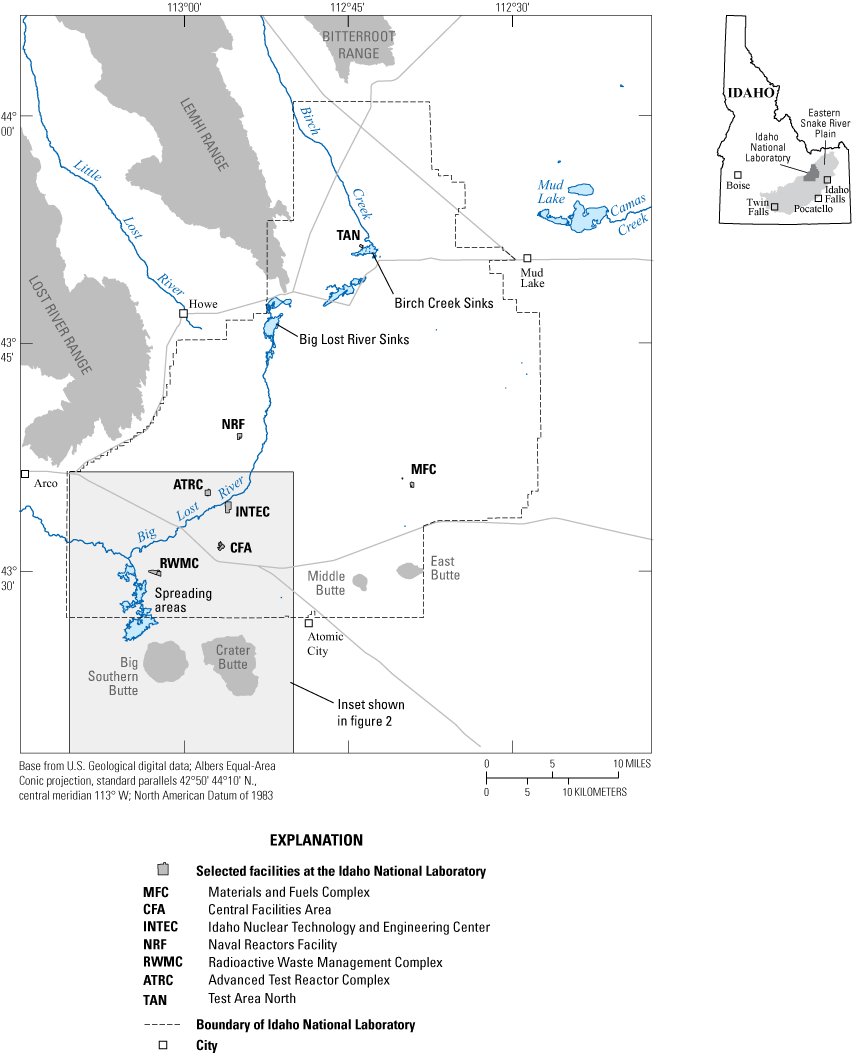
Locations of the Idaho National Laboratory and other select facilities, Idaho.
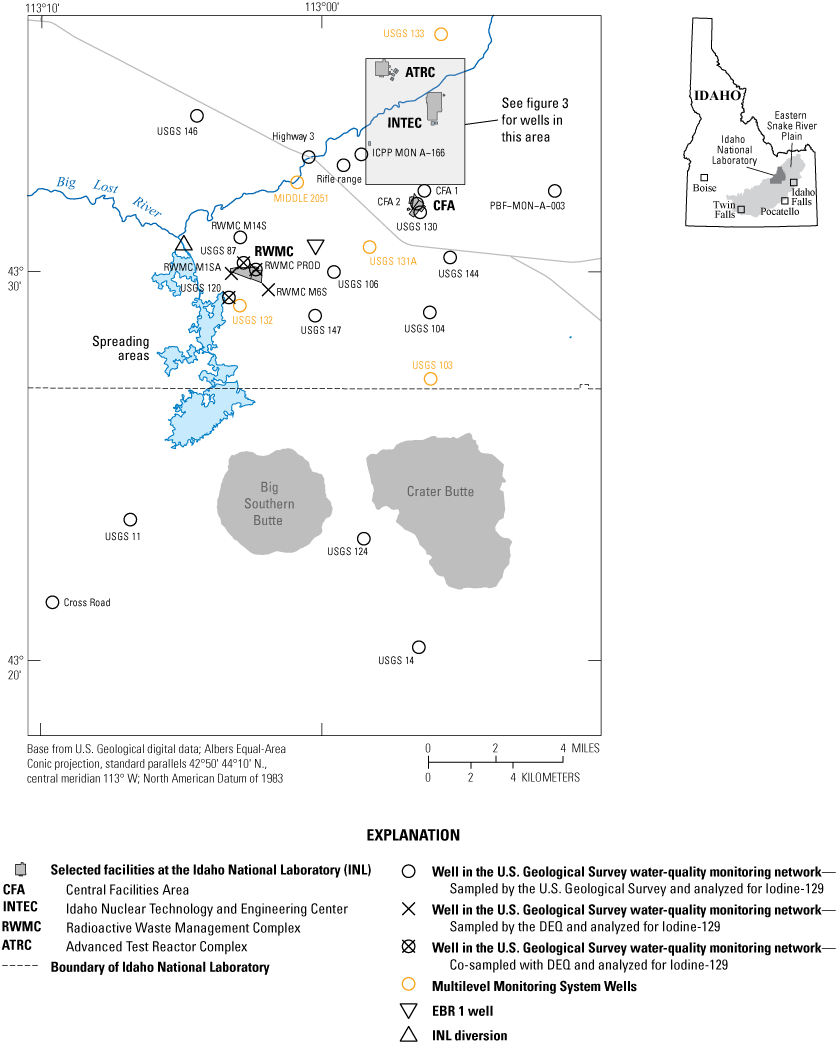
Locations of selected wells at and near the Idaho National Laboratory. DEQ, Department of Environmental Quality; USGS, U.S. Geological Survey.
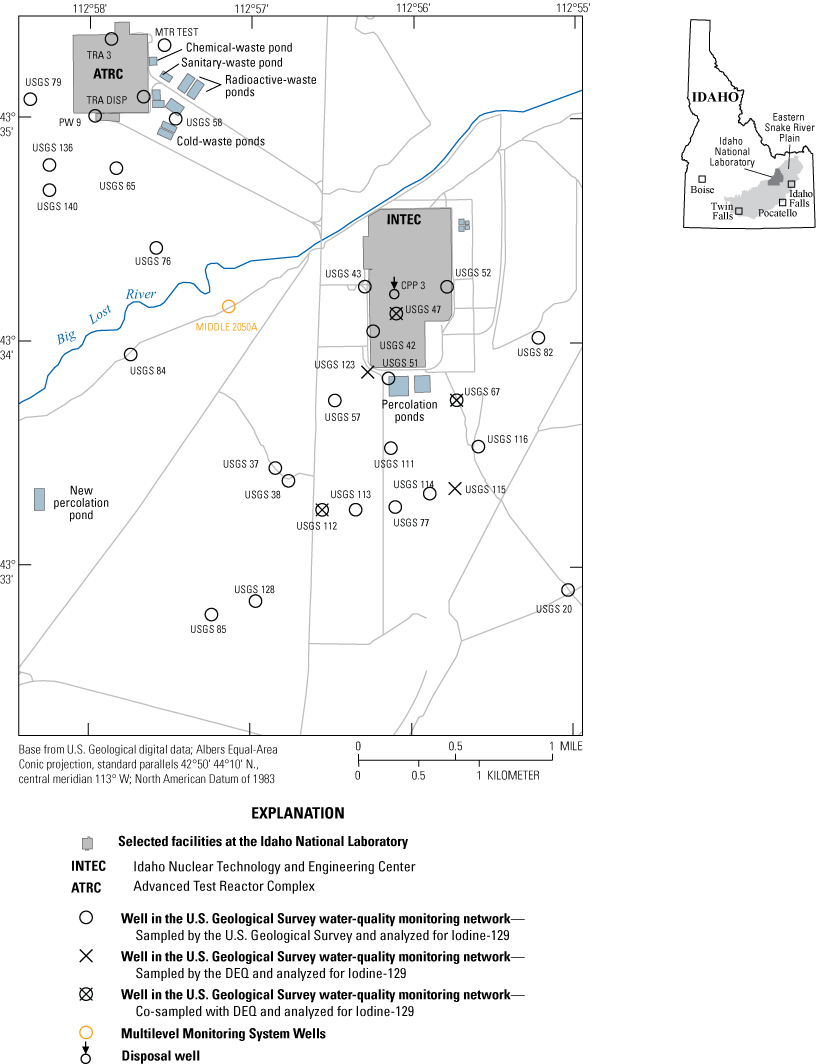
Locations of selected wells at and near selected facilities at the Idaho National Laboratory. DEQ, Idaho Department of Environmental Quality; USGS, U.S. Geological Survey.
Mann and Beasley (1994a) reported that between 1953 and 1990, the wastewater discharged into the injection well and infiltration ponds at the INTEC contained an estimated 0.56–1.18 curies (Ci) of 129I. For scale, results in this study are given as pCi, where 1012 pCi are equivalent to 1 Ci. In 2004, the DOE Idaho Operations Office performed a detailed estimate of wastewater discharge in the area and estimated that a maximum of 0.86 Ci of 129I was discharged to the aquifer through the injection well between 1952 and 1984 (U.S. Department of Energy, 2004, app. D). Furthermore, between 1984 and 1988, about 0.08 Ci of 129I was discharged to the infiltration ponds (Litteer, 1988; Mann and others, 1988, table 2; Litteer and Reagan, 1989). Additionally, from 1958 to 1986, about 0.001 Ci of 129I was released at the INTEC tank farm (Cahn and others, 2006, table 5–2). As a result, traces of 129I may still be in remnant perched zones or sorbed to sediment interbeds beneath the INTEC.
Iodine-129 (129I) in the ESRP aquifer has three potential sources, both natural and anthropogenic: atmospheric deposition, rock weathering, and wastewater disposal (Mann and Beasley, 1994a). The quantities of 129I from atmospheric deposition and rock weathering were considered low but were factored as minor contributors in the ambient local background concentration estimate of 5.4×10−6 pCi/L for the ESRP aquifer in eastern Idaho (Cecil and others, 2003), which is represented by the 95th percentile value from samples from 30 wells and springs considered to be unaffected by disposal practices at the INL. This ambient local groundwater background concentration estimate is used as the baseline for comparison with the samples collected in 2021–22.
The migration of 129I within the hydrological cycle is influenced by several processes that affect its fate and transport. These processes, both chemical and physical, can include chemical reactions controlled by mineral assemblages or microbial communities, adsorption onto sediments that are interbedded with basalt flows at various depths, co-precipitation into iron oxide or calcium carbonate minerals, or interactions with organic matter (Neeway and others, 2019). The fate and transport of 129I within the ESRP aquifer also likely depends on many factors including the redox conditions of the aquifer, sorption onto various aquifer materials, hydrogeological flow constraints, and localized geochemical conditions.
Because of its very long half-life of 15.7 million years (Emery and others, 1972), when 129I is released into the environment, it becomes a permanent addition to the global inventory (Mann and Beasley, 1994a) and can effectively be used as a “stable,” non-hazardous tracer of anthropogenic nuclear activities (Rao, 1997). However, 129I is a carcinogen, and communities downgradient from the INL may be concerned that 129I disposed to groundwater at the INL could be a hazard to their health. The U.S. Environmental Protection Agency maximum contaminant level (MCL) for 129I in drinking water is 1 pCi/L (U.S. Environmental Protection Agency, 2022). The MCL is based on the average concentration of 129I that will yield an annual whole-body dose equivalent to 4 millirem for man-made beta-particle and photon-emitting radionuclides (U.S. Environmental Protection Agency, 2022).
In response to historical releases of anthropogenic 129I, efforts have been taken to monitor its presence in the subsurface environment. The ESRP aquifer, which serves as a vital source of drinking water for 300,000 residents in southern Idaho, has been closely monitored (Idaho Department of Environmental Quality, 2021). To assess potential risks, several agencies have periodically monitored 129I in groundwater from the ESRP aquifer at and downgradient from the INL since 1977. The monitoring efforts done in 1977, 1981, 1986, 1990–91, 2003, 2007, 2010–12, and 2017–18 are summarized by Mann and others (1988); Mann and Beasley (1994b); Bartholomay (2009, 2013); and Maimer and Bartholomay (2019). The definition of the locally determined ambient background level of 129I was most recently evaluated by Cecil and others (2003).
Although select facilities at the INL have historical records that indicate a release of 129I to the aquifer, the historical records at the ATRC facility indicated very minimal 129I was introduced at the ATRC. The evaluation of 129I concentrations in old groundwater at the INL, as determined from low-level tritium analyses, and in groundwater near the ATRC, indicates an incomplete understanding of the concentrations in the wastewater discharged at the ATRC. For example, in 2007, the concentration of 129I in groundwater from well EBR 1 was measured at 8×10−3 pCi/L, about three orders of magnitude higher than the presumed background concentration, indicating that well EBR 1 likely contains wastewater discharged from an upgradient facility, for example, ATRC or INTEC. However, in 1996, the tritium concentration from the same well measured as non-detect, indicating that groundwater from this well is largely devoid of wastewater from facilities such as INTEC or ATRC and probably contains mostly old groundwater (Rattray, 2018). This apparent discrepancy of groundwater with an 129I signature (suggesting wastewater inputs) and a non-detect tritium measurement (suggesting potentially old groundwater) cannot be resolved because of the lack of data to support potential sources of 129I from ATRC. Additional sampling in this area might provide insight into why background concentrations of 129I in groundwater in the southwestern part of the INL are greater than the estimated background groundwater concentrations.
While records of 129I in wastewater discharged at the ATRC are incomplete, available historical records indicate that the 129I concentration in discharged wastewater was approximately 1.3×10−5 pCi/L in 1978 (Batchelder, 1979). However, in 1990–91, an initial evaluation of the Test Reactor Area disposal well (TRA DISP; fig. 3) showed 129I concentrations of 1.1×10−2 pCi/L, four orders of magnitude greater than background concentrations (Mann and Beasley, 1994b). This result indicates that much larger concentrations of 129I were likely discharged in wastewater, or injected via a disposal well, in the past from the ATRC. Consequently, wastewater discharged at the ATRC may be a source of 129I in the groundwater of the southwestern part of the INL.
Groundwater sampling was done to provide an updated assessment of 129I groundwater concentrations across the INL, especially in the southwestern part of the INL, including at or near the ATRC. During 2021–22, the U.S. Geological Survey in cooperation with the DOE and the Idaho Department of Environmental Quality (DEQ) Idaho National Laboratory Oversight Program (INLOP) collected groundwater samples from 64 monitoring wells in the ESRP aquifer, 6 of which are multilevel monitoring systems (MLMS), and one perched well, to determine the concentration of 129I (table 1). All samples in this study were analyzed by accelerator mass spectrometry (AMS) and data are available in Fisher and others (2024) and Idaho Department of Environmental Quality (2022a; 2022b). This sampling is part of an ongoing study to track trends and occurrences of this carcinogenic, long-lived radionuclide in the local environment.
Purpose and Scope
During the fall of 2021 and spring and summer of 2022, the U.S. Geological Survey (USGS) collected groundwater samples from various types of wells, including wells completed in the ESRP aquifer, a well screened in a perched aquifer, and MLMS wells, to evaluate the concentrations of 129I in the ESRP aquifer and perched groundwater. This report describes the 129I concentrations in groundwater and compares these findings with historical data to document concentrations and identify trends in 129I concentrations over time at select wells. USGS personnel collected samples during three events: in October 2021, 29 samples were collected; in April 2022, 22 samples were collected; and lastly, in June 2022, 8 samples were collected from 6 MLMS wells (figs. 2–3). The DEQ INLOP also collected samples at select sites from March to October 2022. For quality assurance, USGS collected seven replicate or quality control (QC) samples sequentially during the three sampling events and evaluated the normalized absolute difference. The DEQ INLOP program collected one replicate QC sample to evaluate quality assurance for this study. All samples collected by USGS and DEQ INLOP for this study were analyzed by AMS at the Purdue Rare Isotope Measurement (PRIME) laboratory, located at Purdue University, West Lafayette, Indiana.
The primary objective of this sampling effort is twofold: first, to investigate the discrepancy of previous results of 129I greater than background at the ATRC, and if this potential source is distinct from the known source at INTEC; and second, to provide an updated assessment of 129I groundwater concentrations across the INL.
Geohydrologic Setting
The INL is in the west-central part of the ESRP. The ESRP is a northeast-trending structural basin about 200 miles (mi) long and 50–70 mi wide (fig. 1). The subsided basin is filled with basaltic lava flows, silicious volcanoclastic sediments, and interbedded sediments from fluvial, alluvial, and aeolian processes (Rodgers and others, 2002; Ackerman and others, 2006; Schusler and others, 2020). The basaltic rocks and sedimentary deposits in the basin combine to form the ESRP aquifer, the main source of groundwater in the basin.
The ESRP aquifer is one of the most productive aquifers in the United States (Maupin and Barber, 2005). Its groundwater typically flows from the northeast to southwest, ultimately discharging to springs along the Snake River, downstream from Twin Falls, Idaho, approximately 100 mi southwest of the INL (fig. 1).
Groundwater movement is both horizontal, through basalt interflow zones, and vertical, through joints and overlapping edges of basalt flows. Factors such as surface-water infiltration, heavy pumping, geohydrologic conditions, and seasonal variations in recharge and discharge rates locally influence groundwater movement (Garabedian, 1986). Recharge to the ESRP aquifer is primarily from infiltration of irrigation water, streamflow, inflow of groundwater from adjacent mountain drainage basins, and precipitation infiltration (Ackerman and others, 2006).
At the INL, depth to water in wells in the ESRP aquifer ranges from about 200 feet (ft) below land surface in the northern part of INL to more than 900 ft below land surface in its southeastern part (for recent water level data, see Treinen and others, 2024). Most of the groundwater flows through the upper 200–800 ft of basaltic rocks (Mann, 1986). Twining and Maimer (2019) reported an estimated transmissivity for the upper part of the aquifer to be 2 to 540,000 feet squared per day. The hydraulic gradient of the aquifer ranges from 2 to 10 feet per mile, with an average of about 4 feet per mile (Twining and Maimer, 2019).
Horizontal flow velocities calculated based on the movement of various constituents in different areas of the aquifer at and near the INL (Robertson and others, 1974; Mann, 1986; Cecil and others, 2000; Plummer and others, 2000; Busenberg and others, 2001), range from 2–26 feet per day. These velocities correspond to a travel time of approximately 50–700 years for water beneath the INL to reach the springs discharging at the terminus of the ESRP near Twin Falls, Idaho (Robertson and others, 1974). Localized groundwater tracer tests at the INL have revealed vertical and horizontal transport rates as high as 60–150 feet per day (Nimmo and others, 2002; Duke and others, 2007). The effective base of the aquifer is about 815 to 1,710 ft below the surface in the western half of the INL and could exceed 1,900 ft in the eastern half (Anderson and Liszewski, 1997).
Previous Investigations
Previous investigations of 129I in water from the ESRP aquifer include those by Barraclough and others (1982), Lewis and Jensen (1985), Mann and others (1988), Mann and Beasley (1994a; 1994b), Cecil and others (2003), U.S. Department of Energy (2004, 2007, 2008, 2012), Hall (2006), Forbes and others (2007), Bartholomay (2009; 2013), and Maimer and Bartholomay (2019). A comprehensive listing of publications by the USGS at the INL is available at https://rconnect.usgs.gov/INLPO/inlpubs-main/ (Fisher, 2022).
One of the first monitoring efforts for 129I at the INL was initiated in April 1977. Results from this sampling indicated concentrations of 129I in 14 wells ranged from 0.9 to 27 pCi/L for statistically positive values (Barraclough and others, 1982, fig. 42). Iodine-129 was identified in wells less than 3 miles from the disposal well In October 1981, concentrations of 129I ranged from 0.05 to 41 pCi/L for statistically positive values (Lewis and Jensen, 1985), where 129I was identified in wells about 6.3 mi from the disposal well. The major difference between the 1977 and 1981 results was that the sample size increased in 1981 from 1 to 4 liters for a fourfold reduction in the reporting level. The increase in sensitivity of analyses (Lewis and Jensen, 1985), along with a more extensive set of wells sampled (20 in 1977 and 32 in 1981) were the primary reasons for the increase in the mapped [spatial] extent of the 129I plume.
In August 1986, 129I concentrations from 20 wells sampled ranged from 0.49±0.12 to 3.6±0.4 pCi/L, with concentrations greater than the reporting level (greater than 3 times the standard deviation; Mann and others, 1988), and 129I migrated about the same distance (6.3 miles) from the disposal well as in 1981. The large decrease in the maximum concentration between 1981 and 1986 was attributed to changes in disposal practices at the INTEC, to the reduction in the mass of 129I in wastewater, and to increased dilution of 129I in the mid-1980s because of a large amount of flow in the Big Lost River (Mann and others, 1988), which is a source of recharge.
Before the 1990–91 data collection, analytical methods using neutron activation were used to quantify 129I in groundwater samples. During 1990–91, Mann and Beasley (1994b) collected samples from 51 wells at and near the INL and analyzed samples using the AMS method, which allowed for an analytical sensitivity that was (2–6 times) more sensitive than neutron activation (Elmore and Phillips, 1987). This increased sensitivity allowed a background 129I concentration of 0.9×10-6±0.2×10−6 pCi/L to be detected in a groundwater sample taken upgradient from the INTEC. The increased sensitivity also resulted in detectable concentrations of 129I downgradient from the INL that were used to calculate groundwater flow velocities of at least 6 feet per day (Mann and Beasley, 1994b). The maximum 129I concentration detected in samples collected during 1990–91 was 3.82±0.19 pCi/L, which was like the maximum concentration detected in 1986; however, mean concentrations from 18 wells sampled in 1986 and 1990–91 decreased from 1.30±0.26 to 0.81±0.19 pCi/L (Mann and Beasley, 1994b). This decrease was attributed largely to declining disposal rates (Mann and Beasley, 1994b).
In 1992, Mann and Beasley (1994a) collected groundwater and surface-water samples to determine background concentrations of 129I. They collected samples from 16 sites that were not likely to have been affected by wastewater disposal at INTEC. These sites encompass a variety of sources, including nine groundwater wells, four springs, and three stream sites that were either on the ESRP or were tributaries flowing onto the plain. The concentrations of 129I in these samples ranged from 0.1±0.1×10−6 to 8.1±0.6×10−6 pCi/L (average of 3.3±0.2×10−6 pCi/L). At the 99-percent confidence level, background concentrations for the 16 sites were estimated to be less than or equal to 8.2×10−6 pCi/L (Mann and Beasley, 1994a).
Cecil and others (2003) reevaluated the background concentrations by analyzing the results of 52 samples collected between 1992 and 1994 from various groundwater and surface-water locations in the ESRP in southeastern Idaho, including the samples collected by Mann and Beasley (1994a). They determined that surface-water samples generally contained larger 129I concentrations than groundwater samples because of anthropogenic fallout and evapotranspiration. To determine the background concentrations, they used a subset of 30 water samples from wells in the aquifer that were in areas considered to be unaffected by disposal practices at the INL. The 95th percentile 129I concentration was 5.4×10−6 pCi/L and was defined as the background concentration, and the 95-percent nonparametric confidence interval was 5.2×10−6 to 10.0×10−6 pCi/L (Cecil and others, 2003). This local ambient background 129I value of 5.4×10−6 pCi/L is used as the baseline concentration for comparison with the samples collected from 2003 forward, including those from this study in 2021−22.
Hall (2006) collected samples from 13 wells downgradient from the INL in 1997 and 1998. Using AMS methods, Hall (2006) determined that concentrations in four of the sites—USGS 11, 14, 124, and 125 (fig. 2)—were greater than estimated background concentrations of Cecil and others (2003). Hall (2006) hypothesized that the 1958 peak 129I concentrations in the ESRP aquifer had already passed these wells. Concentrations of the four sites ranged from 6.1±0.18×10−6 to 7±0.3×10−4 pCi/L, but they were less than concentrations measured in 1991 and 1993.
INL contractors routinely collect 129I from monitoring wells throughout the INL for INL’s Waste Area Group monitoring programs. In 2002, samples were collected from four wells south of INTEC (ICPP wells 1795–1798) from three zones in the aquifer to determine concentrations above and below the H-I interbed, a sedimentary interbed located at depth at INTEC (U.S. Department of Energy, 2004). Concentrations of 129I in well ICPP-1795 increased from 0.34±0.04 pCi/L at 560 ft below land surface to 0.43±0.07 pCi/L at 620 ft below land surface. The three wells farther to the south showed a decrease in 129I concentrations with depth; concentrations in the upper zone ranged from 0.58±0.1 to 0.88±0.08 pCi/L and concentrations in the lower zone ranged from less than the method detection limit (not detected) to 0.33±0.05 pCi/L. The U.S. Department of Energy (2004, fig. 5-5) also presented results from analyses of 49 wells sampled in 2001; concentrations ranged from less than the method detection limit to approximately 0.1 to 1.06 pCi/L. All analyses used gamma spectroscopy methods and all 20 sites sampled were below the MCL of 1 pCi/L (U.S. Department of Energy, 2004, fig. 6-1).
Forbes and others (2007) presented results of 25 wells sampled in 2006 near the INTEC; concentrations ranged from less than the reporting level, or 3 times the standard deviation (3s), to 0.65±0.097 pCi/L in well USGS 67. Analyses of the data collected from 2004–06 at wells around INTEC indicated no discernible change in the concentrations during that time when the analytical uncertainty of the data (+18 percent) was considered (Forbes and others, 2007).
U.S. Department of Energy (2007) presented results of 129I data collected in 2005 and 2006 from 24 wells downgradient from INTEC and the ATRC. Included were several wells south of the INL, along with results from five zones, each from two MLMS wells (Middle 2050A and Middle 2051). Samples were analyzed using the AMS method at the PRIME Lab, and a selected comparison was made to the 2003 USGS data, which is in Bartholomay (2009). Results were used to speculate on the source of 129I in wells around the Radioactive Waste Management Complex (RWMC). Concentrations in most southern wells were greater than background concentrations.
The U.S. Department of Energy (2008) presented results of 129I data collected in 2007 from six zones in one MLMS well (USGS 132). Samples were analyzed using the AMS method at the PRIME Lab, and the results from the six zones ranged from 4±0.13×10−4 to 2.0±0.09×10−3 pCi/L. Results for all six zones were more than two orders of magnitude less than the MCL, but all were greater than the established background concentration of Cecil and others (2003).
Bartholomay (2009) presented results of 129I data collected in 2003 from 36 wells and data collected in 2007 from 36 wells along with concentrations from 31 zones sampled from 6 MLMS wells. Samples were analyzed using the AMS method at the PRIME Lab, and concentrations ranged from 6.6±0.2×10−6 to 1.16±0.04 pCi/L. Their report highlighted concentration increases and decreases through time at various parts of the INL. Decreases were attributed to discontinued disposal and dilution and dispersion in the aquifer. Increases were attributed to variable discharge amounts of wastewater that eventually moved to well locations as a mass of water from a particular disposal period and from the possible movement of remnant concentrations in perched aquifers around INTEC being moved to the ESRP aquifer.
The U.S. Department of Energy (2012) presented results of 129I data collected in 2011 from wells in and around INTEC. Concentrations were less than the MCL at all the monitoring wells, and most of the wells had concentrations below the laboratory detection levels. The highest concentration (0.537 pCi/L) was detected in well USGS 67. Trend plots indicated that 129I concentrations declined significantly from concentrations in the 1980s and 1990s (U.S. Department of Energy, 2012, p.27).
Bartholomay (2013) presented results of 129I data collected from 2010 through 2012 at and downgradient from the INL from 62 wells. Eleven of the 62 wells were MLMS wells and had 25 samples collected from their various zones. Concentrations of 129I in the wells ranged from 1.3±0.5×10−6 to 1.02±0.04 pCi/L and generally decreased near INTEC, relative to previous sampling events. The smallest concentration was collected in a first-time sample from a well near Kimama, Idaho, and first-time samples were also collected from wells near the Naval Reactors Facility (fig. 1), the ATRC, and several MLMS wells (figs. 1 and 3). An elevated concentration of 129I (2.9×10−3) was identified at well USGS 136 immediately to the south of the ATRC and at several nearby MLMS wells.
Maimer and Bartholomay (2019) presented results of 129I concentrations in well samples between 2017–18. This sampling campaign was focused on the area downgradient from INTEC to evaluate trends in 129I concentrations over time. The concentrations of 129I in the aquifer ranged from 1.6±0.1×10−5 to 0.88±0.03 pCi/L. They observed that while wells sampled in 2017–18 near INTEC showed decreasing concentrations compared with historical data, some wells south of the INL boundary showed slightly increased concentrations compared with previous sampling efforts. These increases are attributed to historically variable discharge rates of wastewater that eventually moved to these well locations as a pulse of water from a particular [historical] disposal period. Comparisons of results from the 2021-22 sampling event and results from Maimer and Bartholomay (2019) are given in figure 4.
Methods and Quality Assurance
This study presents results and sample collection methods from two independent entities: the USGS INL Project Office (INLPO) and the Idaho Department of Environmental Quality (DEQ) Idaho National Laboratory Oversight Program (INLOP). Each sampling entity’s sample collection method is detailed as well as established quality assurance practices. Finally, the analytical method and data evaluation are described for all analyses presented in this study.
Sample Collection Methods
Sample collection by the INLPO generally followed the guidelines established by the USGS National Field Manual (U.S. Geological Survey, variously dated) and Bartholomay and others (2021). The DEQ INLOP field staff followed the sampling protocol of the co-sampling organization that led each individual sampling event, either the INLPO or the Idaho Cleanup Project Contractor, Idaho Environmental Coalition, during separate sampling events.
Water samples were mainly collected from wells that are equipped with dedicated submersible pumps. In general, samples are collected from the upper 250 feet (ft) of the aquifer and consist of mixtures of regional valley groundwater, tributary recharge water, old groundwater, and intermittent surface water recharged in or near the INL (Rattray, 2018). In addition to samples taken from standard monitoring wells, samples were also collected from wells that are a part of the multilevel monitoring system (MLMS) and have sampling ports that are at various depth(s) within each well (Twining and others, 2021).
At wells equipped with a dedicated pump, a volume of water, equivalent to at least one borehole volume, is extracted. Throughout the extraction process, parameters such as temperature, pH, dissolved oxygen, and specific conductance are measured using a multiparameter sonde. The monitoring techniques used to quantify the samples are those outlined by Wood (1981), Claassen (1982), and the U.S. Geological Survey (variously dated). Only after the parameters have stabilized are samples collected into corresponding 129I-sample bottles through a disposable 0.45-micrometer filter cartridge that had been pre-rinsed with at least 2 liters of deionized water. A water sample is collected when pH measurements are within +/−0.1 standard units, water temperature is +/−0.2 °C, and specific conductance readings are within 5 percent of each other for three consecutive readings (Bartholomay and others, 2021).
For MLMS wells, samples are collected using a pre-cleaned stainless-steel thief sampling device. This device is lowered into the well to a predetermined depth, either at a sampling port or slightly above the completion depth and filled with water. Upon retrieval of the water to the surface, field parameters are promptly measured and recorded. Once samples are collected, they are then filtered, using a peristaltic pump, through a pre-rinsed disposable 0.45-micrometer filter cartridge and collected into corresponding 129I sample bottles. Samples needed to be filtered to remove particulate matter that could affect the laboratory preparation of the sample targets used in the AMS measurements of 129I (Cecil and others, 2003). No preservation was necessary for the 129I samples, including either chemical preservation or minimum temperature storage accommodations.
The following description is the sample collection method adopted by DEQ INLOP program when sampling with the IEC group. Before sample collection, monitoring wells undergo purging operations and a multiparameter sonde is used to measure various water-quality parameters, including pH, temperature, specific conductance, dissolved oxygen, and turbidity. Purging continues until at least one well volume is purged, and three consecutive measurements fall within specified limits for their particular constituent: pH within ±0.2 units, temperature within ±0.5 degrees Celsius, and specific conductance within ±5 percent of the previous value. Should any of these parameters fail to stabilize, sampling will take place once a three-well volume purge is achieved. Wells with insufficient yields will be purged dry and sampled on the subsequent working day. All 129I samples were sampled using certified clean polypropylene bottles. Samples were delivered to the Environmental Monitoring Laboratory at Idaho State University before analysis at PRIME Laboratory.
Analytical Methods
All USGS and DEQ INLOP collected samples were ultimately sent to the Purdue Rare Isotope Measurement (PRIME) laboratory, located at Purdue University, West Lafayette, Indiana, where 129I concentrations were determined by Accelerator Mass Spectrometry (AMS) methods described on the PRIME Lab website (https://www.physics.purdue.edu/primelab/ams/index.php). These methods were described by Sharma and others (1997, 2000), and quality control requirements were described by Mark W. Caffee, Director of the PRIME Lab (https://www.physics.purdue.edu/primelab/user-information/quality-control.php).
In the laboratory, environmental and quality control (QC) sample aliquots are weighed and spiked with a carrier isotope solution (127I) at a known concentration. These samples then undergo a chemical purification process to produce a silver iodide (AgI) target material that is mixed with an equal volume of Niobium (Nb), which serves as a binder material. The samples are then loaded into aluminum cathodes for AMS analysis. Analyses of the targets produce a ratio of the radionuclide 129I (unknown) to stable 127I (carrier), along with chemistry and mass spectrometry associated uncertainties. Calculated concentrations (as atoms per gram) and analytical uncertainties are derived from the 129I/127I ratios, masses of sample used, carrier mass added, and finally, are converted into units of picocuries per liter (pCi/L).
The PRIME laboratory reported results in pCi/L based on the equation:
whereRs
is the measured sample ratio of 129I atoms to 127I atoms,
Rb
is the ratio of the chemistry blank,
Ws
is the mass of 127I in sample (in mg),
Wc
is the mass of 127I added as carrier (in mg), and
Wt
is the mass of sample aliquot (in mg).
The mass of the 127I carrier, in milligrams, is determined by the PRIME Lab as the product of the carrier volume and carrier concentration. The concentration of iodine in most samples was less than 0.002 milligrams per liter, which was used as the estimate for the concentration for all samples to add the appropriate amount of carrier solutions, except for those that had higher estimated or reported concentrations.
Guidelines for Interpreting Analytical Results
Guidelines for interpreting analytical results are based on an extension of a method proposed by Currie (1984) and given in Mann and Beasley (1994b). Concentrations of 129I are reported with an estimated sample standard deviation, s, obtained by propagating sources of analytical uncertainty from sample preparation and the analytical method. In this report, 129I concentrations less than 3s (three times the standard deviation) are considered less than a “reporting level.” The reporting level is different than the analytical method detection limit, which is based on laboratory procedures (Bartholomay, 2013). Method detection limits for AMS at PRIME were not determined or reported by the laboratory; therefore, the reporting level was used to determine the presence (or absence) of 129I in collected environmental and QC samples.
The laboratory calculations do not consider the concentration of iodine in the sample because the concentration is typically negligible; the concentrations are estimated from previous data at each site, as available (using equations in Rao, 1997). When measured sample 129I/127I ratios are less than the ratio for the carrier blank sample, calculations based on the Rao (1997) equation produce negative results.
Guidelines for Quality Assurance Evaluation
Quality assurance and reproducibility were assessed with replicate samples. The USGS collected seven quality control (QC) replicate samples sequentially to the environmental samples and submitted them for AMS analyses. The DEQ INLOP collected one QC sample and submitted it for AMS analyses.
Williams (1996) provided a detailed explanation of a method defined by Volk (1969) used to determine the statistical equivalency of radiochemical-constituent concentrations in sample-replicate pairs. In this method, statistical equivalence is determined within a specified confidence level. A value for the standard deviate, Z, is calculated, and then the significance level of the result is evaluated (evaluating the level of significance assumes that the sample population is distributed normally). For this report, concentrations of individual constituents in sample-replicate pairs (constituent pairs) were considered equivalent when the results were within two standard deviations of each other. At this confidence level (95-percent), the level of significance, determined from a standard normal probability curve, was 0.05 for a two-tailed test, and it corresponded to a Z-value of 1.96 (Bartholomay, 2013).
The equation used to determine the Z-value was adapted from Volk (1969):
wherex
is the concentration of a constituent in the environmental sample,
y
is the concentration of the same constituent in the sequential replicate sample,
sx
is the standard deviation of x, and
sy
is the standard deviation of y.
When the population is distributed normally, and the standard deviation is known, the analytical results of replicate pairs can be considered statistically equivalent at the 95-percent confidence level if the Z-value is less than or equal to 1.96. When the population is not distributed normally, or an approximation of the standard deviation is used, a Z-value less than 1.96 must be considered as a guide when testing for equivalence (Williams, 1996). The use of equation 2, therefore, is considered a guide to determine if the results of 129I analyses of sample-replicate pairs were equivalent.
Iodine-129 Concentration Evaluation Approach
Kendall Trend Test
The Kendall trend test, a nonparametric method used to identify monotonic trends, was used to evaluate the 129I results from selected wells during six different sample periods between 1990 and 2022 (table 2). This statistical technique is used to ascertain whether a monotonic trend exists, either increasing or decreasing, between the constituent variable “y” and the time variable “t” (Helsel and others, 2020). The subset of wells that had (at least) six 129I results were included in the trend evaluation. The R program, EnvStats, was used to evaluate the change in 129I pCi/L over time, and the results are provided in table 2 (R Core Team, 2024). A confidence level, α, of 0.95 was set, with the alternative hypothesis being no change over time. The Kendall’s S statistic was computed for each pair of 129I (y) sample and sample year (t). In this evaluation, the units of the S-statistic represent the change (either increase or decrease) in pCi/L of 129I over time (in years). If the S-statistic is negative, the trend is decreasing, whereas a positive S-statistic indicates an increasing trend. The temporal trend, which is related to the S-statistic, was determined using the Theil-Sen slope estimator (Sen, 1968; Helsel and others, 2020). Thus, a positive S-statistic corresponds to a positive Theil-Sen slope, and conversely, a negative S-statistic corresponds to a negative Theil-Sen slope. If the p-value is less than 0.05 (α=0.95), the trend estimation is deemed significant. The coefficient of determination (R2) value, which is provided in figure 5 and table 2, serves to quantify the correlation of the variation in one variable (dependent) by variation in the other variable (independent).
Kriging Interpolation
The areal distribution of the 129I concentration was created using a kriging (quantile kriging) interpolation method used by Fisher and others (2021) and the ObsNetQW package in the R programming language (Fisher, 2021; R Core Team, 2024). Quantile kriging was applied to 129I concentrations from water samples collected in 2021–22, and negative values were set equal to zero.
Kriging estimates of 129I concentrations, as the natural log in nanograms/liter, are predicted at points on an evenly spaced grid, with a spacing of 100 meters, within the active part of the interpolation grid that is defined within the figure extent. The kriging analysis results in a predicted average concentration value for each cell on the grid. Contour lines of the prediction grid were created and overlain on the measured data. The concentration contours are spaced at 2 natural log units. Kriging predictions are evaluated by graphing a sample variogram, which estimates the spatial correlation of a constituent concentration, that is, the concentration variance by lag distance, in miles. Estimates of variance between values of predicted 129I concentrations separated by varying lag distances are allocated into lag bins and averaged within each bin (Fisher and others, 2021). The lag-bin settings for iodine vary spatially because of multiple plume sources caused by facilities coalescing.
The sample variance is compared with the theoretical (predicted) variance to determine the spatial correlation. The numerical values assigned to the symbols in figure 6B represent the number of theoretical (sampled) data pairs that were evaluated for the quantile kriging application to the 129I dataset. The variogram is drawn as an estimated curve and shows variance lags in distance (miles). Equations used for this method are described in detail in Fisher and others (2021). The variograms for 129I showed good correlation, or model fit (R2=0.66). The kriging interpolation method, applied to 129I samples collected during 2021 and its corresponding variogram, suggest that this method of interpretation is appropriate for estimating the areal concentration distribution. The limitations of quantile kriging and the corresponding estimates of variance in the context of this dataset, could arise from large local variability in constituent concentrations, potentially overlapping plumes, or spatial proximity of sampling sites (Fisher and others, 2021).
Concentrations of Iodine-129 in the Eastern Snake River Plain Aquifer
The USGS and DEQ INLOP collected samples from 64 monitoring wells, including 1 perched groundwater well and 6 MLMS wells in and around the INL between October 2021 and June 2022 (table 1). The DEQ INLOP program also collected samples from 10 wells in the ESRP aquifer between March–October 2022. All samples collected by the USGS and DEQ INLOP had 129I concentrations that were greater than the reporting level of 3s and exceeded the estimated background level of 5.4×10−6 pCi/L for the ESRP aquifer (Cecil and others, 2003), except one sample collected by DEQ had a negative 129I concentration (non-detect) value. Of the remaining samples above the reporting level (3s), concentrations ranged from 8.68±0.64×10−6 pCi/L at TRA 3 to 0.968±0.023 pCi/L at USGS 67 (table 1). All samples collected for this current study had concentrations less than the U.S. Environmental Protection Agencies’ maximum contaminant level (MCL) of 1 pCi/L (U.S. Environmental Protection Agency, 2022).
An analysis of 129I sample-replicate pairs and calculated Z-values for USGS and DEQ collected QC samples are reported in table 1. Results for six of the eight sample-replicate pairs had Z-values less than or equal to 1.96 and are considered statistically equivalent. The two sample-replicate pairs that were not within the 1.96 threshold (95-percent confidence interval) had Z-values of 2.2 and 3.1, with results in the 10−4 and 10−5 pCi/L concentration range; however, these sample-replicate pairs were within the 99 percent confidence interval (3-sigma) for reproducibility. Results for the sample-replicate pairs generally indicated that the sample collection and laboratory procedures used were appropriate for the analytical data obtained. However, with the criteria used in this study here, there may be some concern about statistically significant values being able to be reproduced at very low concentration levels, such as for USGS 79 (table 1).
Table 1.
Iodine-129 concentrations in groundwater at the Idaho National Laboratory and vicinity, 2021–22.[Site information is available from the U.S. Geological Survey National Water Information System at https://waterdata.usgs.gov/nwis (U.S. Geological Survey, 2024). Water-quality data are available in Fisher and others (2024) and Idaho Department of Environmental Quality (2022a; 2022b). See figures 2 and 3 for well locations. Uncertainties are 1-sigma. Dates are given in month/day/year. Abbreviations: ft, foot; 129I, Iodine-129; pCi/L, picocurie per liter; —, no data available; USGS, U.S. Geological Survey; INL, Idaho National Laboratory; DEQ, Department of Environmental Quality]
Historically, concentrations of 129I in select wells generally decreased over time. The average concentration of 129I in a subset of wells that were sampled by the USGS during all six sample periods between 1990 and 2022 decreased from 1.15 pCi/L in 1990–91 to 0.15 pCi/L in 2021–22 (table 2). The average 129I concentration, in pCi/L, of the same 15 wells sampled during six different sample periods are: 1.15 (1990–91), 0.270 (2003), 0.264 (2007), 0.173 (2011–12), 0.168 (2017–18), and 0.149 (2021–22; table 2). These decreases are attributed to the discontinuation of the disposal of 129I in wastewater and to dilution and dispersion in the aquifer (Bartholomay, 2013). Variable increasing and decreasing concentrations in samples between collection periods at individual wells may be attributed to changes in surface-water recharge either from flow in the Big Lost River or from local snowmelt and anthropogenic sources. To gain additional insight into 129I trends over time, two additional well trends were evaluated because of sampling by DEQ, USGS 47 and USGS 67; however, these wells are not included in the average concentrations over time because they were not sampled during every sampling event.
Although most wells sampled in 2021–22 near INTEC showed decreases in concentrations compared with data collected previously (fig.4), some wells south and east of the Central Facilities Area (CFA) (CFA-1, CFA-2) and south of the INL (USGS 11, USGS 14, and USGS 124) showed small increases (table 2). These slight increases are attributed to variable rates of wastewater release, which eventually migrated to these well locations in the form of a water pulse from a specific disposal period. This movement was facilitated by surface-water flow from the Big Lost River or artificial recharge at individual sites caused by wastewater disposal activities.
Between the two most recent sampling events in 2017–18 and 2021–22, USGS 14, south of the INL boundary (fig. 2), showed the largest increase of 122 percent in 129I concentrations (table 2). On the other hand, USGS 116 showed the largest percentage decrease during the period, with a decrease of 60 percent. In this study, the highest concentration of 129I was at USGS 67, southeast of the INTEC (table 1; fig. 3) in 2021–22. This concentration was 0.968 pCi/L, an increase of 0.091 pCi/L or 10 percent from the 2017–18 study (Maimer and Bartholomay, 2019).
Table 2.
Concentrations of Iodine-129 in water from selected wells near Idaho National Laboratory and its vicinity, 1990–2022.[Water-quality data are available in Fisher and others (2024) and Idaho Department of Environmental Quality (2022a, 2022b). See figures 2 and 3 for well locations. Concentrations and analytical uncertainties are in picocuries per liter (pCi/L). All samples were measured by Accelerator Mass Spectrometry at IsoTrace Lab, 1990–91, or Prime Lab, 2003, 2007, 2011–12, 2017–18, 2021–22); uncertainties are 1-sigma (1s). See Mann and Beasley(1994b); Bartholomay (2009, 2013)5; Maimer and Bartholomay (2019) for more information. % change, percent change between 2017–18 and 2021–22. Abbreviations: ID, identification; %, percent; ±, plus or minus; R2, coefficient of determination; NS, not sampled; —, no data available; NC, no change; USGS, U.S. Geological Survey; Avg. conc., Average concentration]
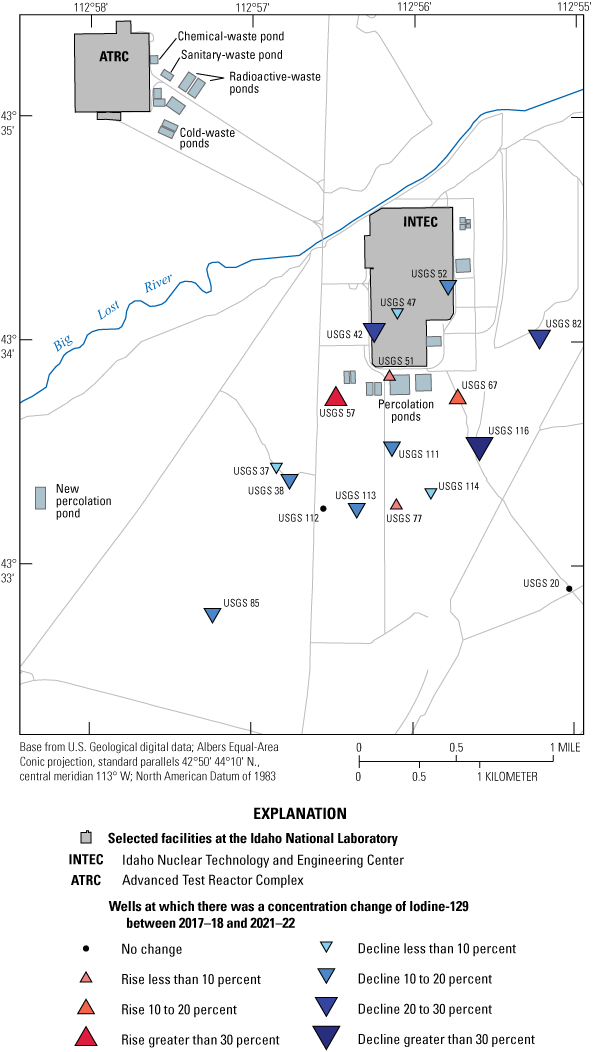
Change in Iodine-129 concentrations between select samples collected between 2017–18 and 2021–22 at select wells at the Idaho National Laboratory. pCi/L, picocuries per liter; R2; coefficient of determination.
Wells USGS 20 and 104 are the only two wells with long-term measurements that show an increasing trend (table 2), although USGS 104 does have one measurement outside of the 2-sigma confidence interval. Maimer and Bartholomay (2019) also evaluated trends and showed USGS 20 had an increasing trend. Iodine-129 concentrations have fluctuated over the 32-year sampling period, showing increases and decreases at several wells, likely depending on disposal practices, surface-water recharge, and localized flow dynamics (fig. 5). Although the trends at wells USGS 20 and 104 are classified as increasing, they are not statistically significant according to the Kendall Trend (Thiel-Sen slope) evaluation (table 2). Of the 17 wells evaluated for trend over time (using DEQ data for USGS 47 and USGS 67), a large majority (13) show a decreasing trend, and 8 of these wells had statistically significant decreasing trends. The remaining two wells, USGS 111 and USGS 116, did not have a statistically discernible trend over this period.
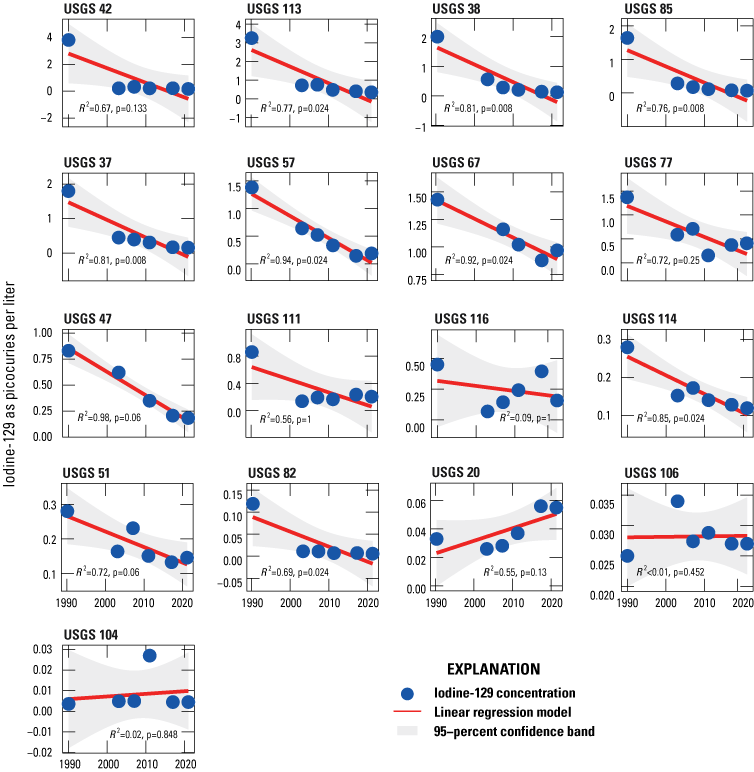
Concentration trends of long-term Iodine-129 data in wells at Idaho National Laboratory. USGS, U.S. Geological Survey.
Mirus and others (2011) indicated that streamflow from the Big Lost River provides recharge to the shallow, intermediate, and deep perched zones within about 150 m of the river and suggested other perched water dynamics, including local snowmelt and anthropogenic sources (such as leaky pipes and drainage ditches), contribute to the recharge of shallow and intermediate perched zones throughout much of INTEC. Water flowed in the Big Lost River channel, below the INL diversion, during the spring of 2017–19; however, very little water flowed below the diversion in 2020; and no water flowed below the diversion between 2021 and 2022 (Bartholomay and others, 2020; Treinen and others, 2024).
Although most of the wells south of INTEC showed a decrease in 129I concentrations between the 2017–18 and 2021–22 studies, some showed increasing concentrations (USGS 51, USGS 57, USGS 67, and USGS 77). Davis and others (2015) suggested that the aquifer southeast of INTEC has lower transmissivity, locally, which may account for the slower wastewater movement and the consequent increase in concentrations at select wells in this area in 2021–22. Previous studies have evaluated the mobility of different isotopes in the ESRP and found that 129I is lessened by sorption in fine-grained material and sediment layers at depth resulting in slower (attenuated) movement in the system (Mann and others, 1988; Beasley and others, 1998). During higher-than-average precipitation years, surface-water recharge may mobilize constituents in perched groundwater and move them into the aquifer, which can result in increasing concentrations of 129I in the aquifer between discrete sampling periods.
By expressing the spatial distribution of 129I as the natural log (in nanogram/L), the 129I plume generally follows the groundwater flow south of INTEC (fig. 6). This plume direction is consistent with observations from previous studies (Bartholomay, 2013; Maimer and Bartholomay, 2019). This spatial distribution method also aids in identifying a lower concentration plume that originates from the ATRC. Despite the late 1970s records (Batchelder, 1979) indicating extremely low concentrations (10-6 pCi/L) being discharged at the ATRC infiltration ponds, a distinct low-level 129I plume is observed, separate from the known plume at INTEC. The location of the plume downgradient from ARTC is based on 129I concentration results greater than 0.00035 pCi/L obtained in 2021–22 from wells USGS 58, 65, 136, and 140, and well PW–9 (table 1), directly south and southwest of the ATRC (figs. 3 and 6). An elevated 129I concentration of 2.9×10−3 pCi/L was identified from well USGS 136 immediately to the south of the ATRC by Bartholomay (2013), and an identical value was obtained from sampling in 2021 (table 1). The targeted sampling campaign at the wells south of ATRC, along with the high-precision, low-level detection capability of AMS methods, offers a more comprehensive understanding of this low-level 129I plume.
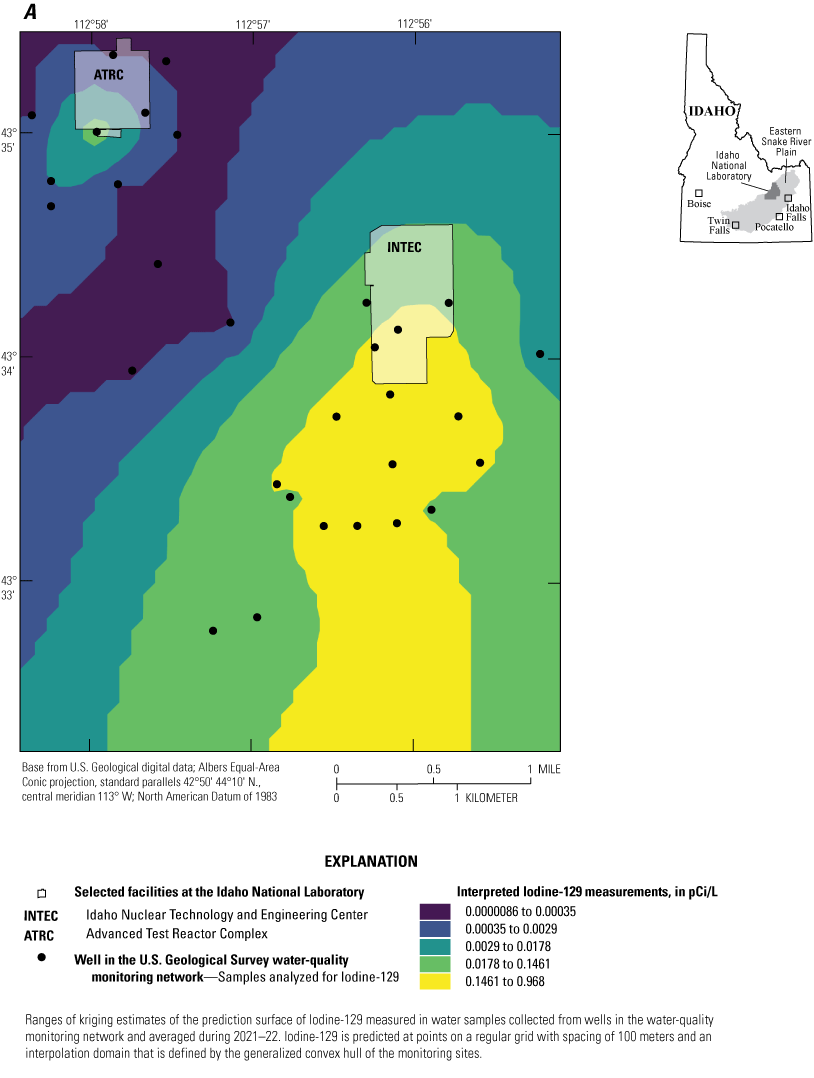
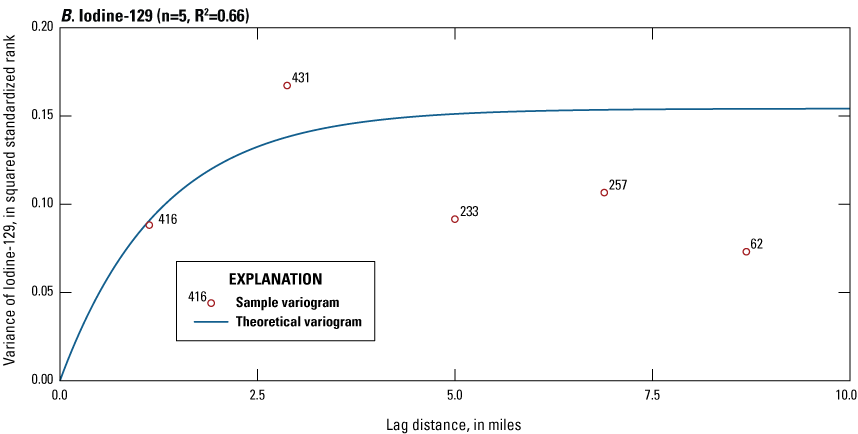
(A) Kriging estimates of the Iodine-129 prediction surface in water overlain on the empirical concentrations from wells at and near the Advanced Test Reactor Complex (ATRC) and the Idaho Nuclear Technology and Engineering Center (INTEC). (B) Variogram analysis of Iodine-129 transformed into standardized rank space for wells at the Idaho National Laboratory, Idaho. USGS, U.S. Geological Survey; pCi/L, picocuries per liter; R2, coefficient of determination.
By grouping the 129I results according to the geographical regions associated with facilities as defined in table 1, we can compare the median concentrations of 129I at various geographic areas such as around INTEC, ATRC, CFA, RWMC, and the area south of the INL facilities. Boxplots are used to display the log (pCi/L) concentrations in each major area, displaying the median concentration (bar), and the maximum distribution, interquartile range, and minimum distribution of each location-defined dataset (fig. 7). The median 129I concentrations vary from 0.2315 pCi/L at INTEC (n=23) to 0.0027 pCi/L south of the INL area (n=6). Wells near INTEC exhibit the highest overall median 129I concentration and the narrowest distribution, reflecting the known source and temporal migration of 129I from this facility. Median 129I concentrations at ATRC facility wells are marginally higher than the median concentrations south of the INL. All observed, 129I concentrations across all regions and facilities exceed the background 129I concentration of 5.4×10−6 pCi/L (Cecil and others 2003) for groundwater, suggesting that the groundwater quality at these locations as well as downgradient locations have been adversely affected.
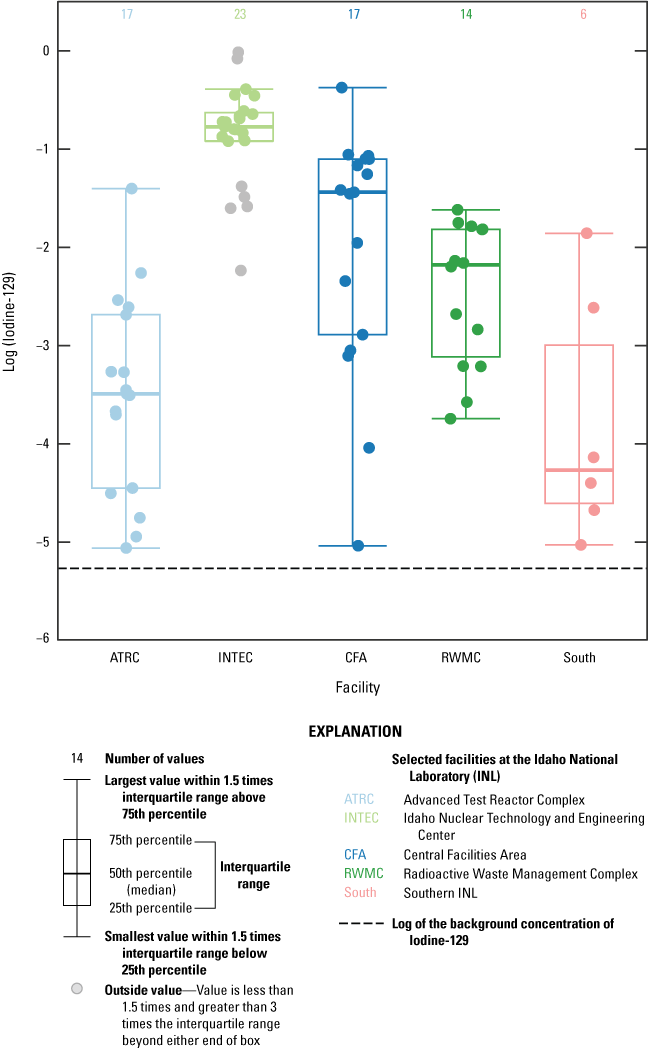
Median concentrations of Iodine-129 [log pCi/L] near select facilities in the Idaho National Laboratory . pCi/L, picocuries per liter.
Multilevel Monitoring System Well Discussion
The INL groundwater monitoring network used a network of MLMS wells to acquire water-quality data at various depths to evaluate the vertical distribution of constituents in the ESRP aquifer (Twining and others, 2021). This study focused on eight zones from six MLMS wells (table 3) near various facilities and along the ephemeral Big Lost River channel. Sampling these various vertical zones at defined port depths allows 129I to be analyzed at various depths within the ESRP aquifer and may inform 129I mobilization and or migration behavior from sources, both at the surface and at depth.
USGS 103 zone 1 is the deepest sampling zone at this well and is near the southern boundary of the site, about 8 miles from INTEC (fig. 2). High tritium concentrations in the deepest sampling zones of this well indicate that wastewater from INTEC has affected these zones (Rattray, 2023). The concentration of 129I in this well (table 3) increased between 2012 and 2022 and is four orders of magnitude greater than groundwater background concentration (table 3; Cecil and others, 2003). Assuming 129I wastewater was discontinued in 1984 (Mann and Beasley, 1994b) and the groundwater velocity (1.3 meter per day) and age (26 years) determined by Busenberg and others (2001) are appropriate, the 129I signature in groundwater originating from injected water at INTEC could reach USGS 103 around 2010, which is consistent with previous 129I results from 2012.
USGS 131A is southwest of CFA (fig. 2). The two shallower zones sampled at USGS 131A, zone 12 and zone 8, had only slightly decreasing 129I concentrations between 2012 and 2022; however, these are within 1-sigma (table 3). Geochemical modeling suggests water in these zones travels south from sources at INTEC and has wastewater signatures including high tritium and high chloride concentrations (Rattray, 2023) and most likely travels south from sources at INTEC.
Well USGS 132 is near the spreading areas and RWMC, and zone 14 is the second sampling zone below land surface at this well (fig. 2). The 129I concentration increased in this zone between 2012 and 2022 (table 3). Geochemical mass-balance modeling suggests the sources of recharge to this zone are from surface-water from the Big Lost River when it inundates the spreading areas as well as groundwater from the tributary valley. A geochemical mass-balance modeling study used the tritium and chloride concentrations from this zone as evident that wastewater originating from ATRC travels south to affect this well (Rattray, 2023).
USGS 133 is the northernmost MLMS well; it is north of INTEC and northeast of ATRC (fig. 2). The most recent 129I concentration measurement at USGS 133 zone 10 (the uppermost sampling zone) was an order of magnitude higher than what was measured in 2012 (table 3). Given the location of this well and that the latest measurement at this sampling zone is greater than the groundwater background of Cecil and others (2003), the source of 129I at this well is not well understood. More data would be needed to determine if an air-born source of 129I from ATRC or INTEC was contributing 129I to the soil and, ultimately, the groundwater.
The 129I concentration of 1.13×10−5 pCi/L observed at Middle 2050A zone 15 (table 3; fig. 3), which represents the uppermost sampling zone within the well, was above the background concentration for the ESRP aquifer defined by Cecil and others (2003) and was higher than that measured in 2012 (Bartholomay, 2013). Results from geochemical mass-balance modeling, the proximity of the well to the Big Lost River, and stable isotope signatures all indicate the water in this uppermost zone is likely affected significantly by surface-water recharge, with a small percentage of wastewater coming from ATRC or INTEC (Rattray, 2023).
Two sample zones at Middle 2051, zone 3 and zone 9, had very similar concentrations between 2012 and 2022 (fig. 2; table 3). These two Middle 2051 zones represent deeper groundwater near the Big Lost River channel and south of ATRC, in the direction of downgradient groundwater flow. Geochemical mass-balance modeling has shown that wastewater [using chemical signatures] in these deep sampling zones supports the concept that groundwater moves downward along groundwater flow paths (Rattray, 2023). The 129I concentrations are about one order of magnitude greater in the deeper zone 3 than in the shallower zone 9. The observed 129I concentrations in this well may be because 129I bearing wastewater was released at ATRC (Batchelder, 1979).
Table 3.
Concentrations of Iodine-129 in groundwater from select multilevel monitoring wells at Idaho National Laboratory and vicinity, Idaho, 2012–22.[Site information is available from the U.S. Geological Survey National Water Information System (NWIS) at https://waterdata.usgs.gov/nwis (U.S. Geological Survey, 2024). Water-quality data are available in Fisher and others (2024). See figures 2 and 3 for well locations. Concentrations and analytical uncertainties are in picocuries per liter (pCi/L); uncertainties are 1-sigma (1s). Dates given in month/day/year. Abbreviations: MLMS, multilevel monitoring systems; no., number; ft, foot; 129I, Iodine-129; ±, plus or minus; USGS, U.S. Geological Survey]
Summary
From 1953 to 1988, the Idaho National Laboratory (INL) in southeastern Idaho generated wastewater containing approximately 0.94 curies of Iodine-129 (129I). This wastewater was a by-product of fuel reprocessing activities at the Idaho Nuclear Technology and Engineering Center (INTEC) at the INL. Most of the wastewater was discharged directly into the eastern Snake River Plain (ESRP) aquifer through a deep disposal well until 1984; however, some wastewater was also discharged into unlined infiltration ponds or leaked from distribution systems below INTEC. There is historical documentation that a small amount of 129I was also discharged at the Advanced Test Reactor Complex (ATRC) as well.
The U.S. Geological Survey (USGS) monitored 129I in the ESRP aquifer at the INL in 1977, 1981, 1986, 1990–91, 2003, 2007, 2010–12, and 2017–18 before sample collection in 2021–22. Additional sampling was done in the 1990s to help determine an estimated local ambient background concentration for 129I in the ESRP of 5.4×10−6 picocuries per liter (pCi/L). Recently collected 129I concentrations in the ESRP aquifer at the INL are compared to the local ambient background concentration for the aquifer and to the U.S. Environmental Protection Agency’s maximum contaminant level of 1 pCi/L.
In 2021–22, the USGS, in cooperation with the U.S. Department of Energy and the Idaho Department of Environmental Quality (DEQ) INL oversight program collected groundwater samples from 64 monitoring wells to determine 129I concentrations in them. Seven quality control samples were collected by the USGS, and one quality control sample was collected by DEQ during this study. Concentrations of 129I above the reporting level in the aquifer ranged from 8.68±0.64×10−6 pCi/L in the TRA 3 well at the ATRC to 0.968±0.023 pCi/L in well USGS 67 south of INTEC. A focused sampling effort around the ATRC was done to evaluate 129I concentrations in the aquifer near this facility because less frequent sampling has been done there previously. The low-level analytical methods and spatial interpolation methods elucidated an independent low-level 129I plume at ATRC, although detailed information could be gained by additional sampling.
The average concentration of 15 wells sampled by the USGS during six different sample periods decreased from 1.15 pCi/L in 1990–91 to 0.145 pCi/L in 2021–22. Evaluating a Kendall Trend test of 17 wells sampled by the USGS and DEQ, sampled from 1990 to 2022, showed that concentration trends are decreasing in 13 wells (statistically significant in eight), increasing in 2 wells, and no trend was identified in 2 wells.
Monitoring multilevel monitoring (MLMS) wells for this study were compared to monitoring results last collected in 2012 from the same sampling zones. 129I did not consistently increase or decrease in these sampling zones between sampling periods. The uppermost sampling zones (closest to the land surface) did show an impact from variable surface-water recharge between 2012 and 2021–22. The deeper zones sampled showed very little change in concentrations between sampling periods, evidence that wastewater from facilities upgradient is continuing to affect these wells.
Decreasing trends in 129I over time are attributed to 129I no longer being disposed of in wastewater and to 129I’s dilution and dispersion throughout the aquifer. Variable [increasing or decreasing] concentrations between sample collection periods at individual wells may be attributed to variable surface-water recharge either from temporally controlled flow in the Big Lost River or from local snowmelt or wastewater sources. Surface-water recharge can either dilute groundwater wastewater signatures, remobilize water from past disposal periods, or make adsorbed 129I from fine-grained material or interbedded sedimentary layers available for transport.
Overall, this recent sampling effort provides a snapshot of 129I concentrations in 64 monitoring wells at the INL, including one perched groundwater well and six multilevel monitoring wells. With this data, it became possible to statistically evaluate the long-term 129I concentration trends at a subset of wells, with many showing decreasing concentrations. The spatially focused sampling effort has also identified a previously not defined low-level 129I plume that affects long-term water quality near and downgradient from the ATRC.
References Cited
Ackerman, D.J., Rattray, G.W., Rousseau, J.P., Davis, L.C., and Orr, B.R., 2006, A conceptual model of ground-water flow in the eastern Snake River Plain aquifer at the Idaho National Laboratory and vicinity with implications for contaminant transport: U.S. Geological Survey Scientific Investigations Report 2006–5122 (DOE/ID–22198), 62 p. [Also available at https://doi.org/10.3133/sir20065122.]
Anderson, S.R., and Liszewski, M.J., 1997, Stratigraphy of the unsaturated zone and the Snake River Plain aquifer at and near the Idaho National Engineering Laboratory, Idaho: U.S. Geological Survey Water-Resources Investigations Report 97–4183, 65 p. [Also available at https://pubs.er.usgs.gov/publication/wri974183.]
Barraclough, J.T., Lewis, B.D., and Jensen, R.G., 1982, Hydrologic conditions at the Idaho National Engineering Laboratory, Idaho—Emphasis: 1974–1978: U.S. Geological Survey Water Supply Paper 2191, 52 p. [Also available at https://doi.org/10.3133/wsp2191.]
Bartholomay, R.C., 2009, Iodine-129 in the Snake River Plain aquifer at and near the Idaho National Laboratory, Idaho, 2003 and 2007: U.S. Geological Survey Scientific Investigations Report 2009–5088, 28 p. [Also available at https://doi.org/10.3133/sir20095088.]
Bartholomay, R.C., 2013, Iodine-129 in the eastern Snake River Plain aquifer at and near the Idaho National Laboratory, Idaho, 2010–12: U.S. Geological Survey Scientific Investigations Report 2013–5195 (DOE/ID-22225), 22 p. [Also available at https://doi.org/10.3133/sir20135195.]
Bartholomay, R.C., Maimer, N.V., Rattray, G.W., and Fisher, J.C., 2020, An update of hydrologic conditions and distribution of selected constituents in water, Eastern Snake River Plain Aquifer and perched groundwater zones, Idaho National Laboratory, Idaho, emphasis 2016–18: U.S. Geological Survey Scientific Investigations Report 2019–5149 (DOE/ID-22251), 82 p. [Also available at https://doi.org/10.3133/sir20195149.]
Bartholomay, R.C., Maimer, N.V., Wehnke, A.J., and Helmuth, S.L., 2021, Field methods, quality-assurance, and data management plan for water-quality activities and water-level measurements: U.S. Geological Survey Open-File Report 2021–1004, 76 p. [Also available at https://doi.org/10.3133/ofr20211004.]
Batchelder, H.M., 1979, Radioactive waste management information for 1979 and Record-to-Date,: U.S. Department of Energy, IDO-10057(79), prepared by EG&G Idaho, Inc., 91 p. [Also available at https://inldigitallibrary.inl.gov/PRR/81246.pdf.]
Beasley, T.M., Dixon, P.R., and Mann, L.J., 1998, 99Tc, 236U, and 237Np in the Snake River Plain aquifer at the Idaho National Engineering and Environmental Laboratory, Idaho Falls, Idaho: Environmental Science and Technology, v. 32, no. 24, p. 3875–3881. [Also available at https://doi.org/10.1021/es980390d.]
Busenberg, E., Plummer, L.N., and Bartholomay, R.C., 2001, Estimated age and source of the young fraction of ground water at the Idaho National Engineering and Environmental Laboratory: U.S. Geological Survey Water-Resources Investigations, Report 2001–4265 (DOE/ID-22177), 144 p. [Also available at https://doi.org/10.3133/wri014265.]
Cahn, L.S., Abbott, M.L., Keck, J.F., Martian, P., Schafer, A.L., and Swenson, M.C., 2006, Operable unit 3-14 tank farm soil and groundwater remedial investigation/baseline risk assessment: U.S. Department of Energy, Idaho Operations Office Publication, DOE/NE-ID-11227, rev. 0, [variously paged]. [Also available at https://idahoenvironmental.com/ARIR/default.]
Cecil, L.D., Hall, L.F., and Green, J.R., 2003, Reevaluation of background iodine-129 concentrations in water from the Snake River Plain aquifer, Idaho, 2003: U.S. Geological Survey Water-Resources Investigations Report 03–4106 (DOE/ID-22186), 18 p. [Also available at https://doi.org/10.3133/wri034106.]
Cecil, L.D., Welhan, J.A., Green, J.R., Grape, S.K., and Sudicky, E.R., 2000, Use of chlorine-36 to determine regional-scale aquifer dispersivity, eastern Snake River Plain aquifer, Idaho/USA: Nuclear Instruments and Methods in Physics Research, Section B: Beam Interactions with Materials and Atoms, v. 172, p. 679–687. [Also available at https://doi.org/10.1016/S0168-583X(00)00216-0.]
Claassen, H.C., 1982, Guidelines and techniques for obtaining water samples that accurately represent the water chemistry of an aquifer: U.S. Geological Survey Open-File Report 82–1024, 49 p. [Also available at https://doi.org/10.3133/ofr821024.]
Davis, L.C., Bartholomay, R.C., Fisher, J.C., and Maimer, N.V., 2015, Water-quality characteristics and trends for selected wells possibly influenced by wastewater disposal at the Idaho National Laboratory, Idaho, 1981–2012: U.S. Geological Survey Scientific Investigations Report 2015–5003 (DOE/ID-22233), 105 p., 5 app. [Also available at https://doi.org/10.3133/sir20155003.]
Duke, C.L., Roback, R.C., Reimus, P.W., Bowman, R.S., McLing, T.L., Baker, K.E., and Hull, L.C., 2007, Elucidation of flow and transport processes in a variably saturated system of interlayered sediment and fractured rock using tracer tests: Vadose Zone Journal, v. 6, no. 4, p. 855–867. [Also available at https://doi.org/10.2136/vzj2006.0102.]
Elmore, D., and Phillips, F.M., 1987, Accelerator mass spectrometry for measurement of long-lived radioisotopes: Science, v. 236, p. 543–550. [Also available at https://doi.org/10.1126/science.236.4801.543.]
Emery, J.F., Reynolds, S.A., Wyatt, E.I., and Gleason, G.I., 1972, Half-lives of radionuclides—IV: Nuclear Science and Engineering, v. 48, no. 3, p. 319–323, accessed February 27, 2024, at https://doi.org/10.13182/NSE72-A22489.
Fisher, J.C., 2021, ObsNetQW—Assessment of a water-quality aquifer monitoring network: U.S. Geological Survey software release. [Also available at https://doi.org/10.5066/P9X71CSU.]
Fisher, J.C., 2022, inlpubs—Bibliographic information for the U.S. Geological Survey Idaho National Laboratory Project Office: U.S. Geological Survey software release, R package, accessed May 1, 2023, at https://doi.org/10.5066/P9I3GWWU.]
Fisher, J.C., Bartholomay, R.C., Rattray, G.W., and Maimer, N.V., 2021, Optimization of the Idaho National Laboratory water-quality aquifer monitoring network, southeastern Idaho: U.S. Geological Survey Scientific Investigations Report 2021–5031 (DOE/ID-22252), 63 p. [Also available at https://doi.org/10.3133/sir20215031.]
Fisher, J.C., Trcka, A.R., and Treinen, K.C., 2024, Datasets for the U.S. Geological Survey—Idaho National Laboratory groundwater and surface-water monitoring networks, v1.1: U.S. Geological Survey data release, https://doi.org/10.5066/P9UWRYR4.
Forbes, J.R., Ansley, S.L., and Leecaster, M., 2007, INTEC groundwater monitoring report (2006): U.S. Department of Energy, Idaho Operations Office Publication, DOE/ID-11301, rev. 0, 134 p. [Also available at https://idahoenvironmental.com/ARIR/default.]
Garabedian, S.P., 1986, Application of a parameter-estimation technique to modeling the regional aquifer underlying the eastern Snake River Plain, Idaho: U.S. Geological Survey Water Supply Paper 2278, 60 p. [Also available at https://pubs.usgs.gov/wsp/2278/report.pdf.]
Hall, L.F., 2006, Concentrations of selected trace metals, common ions, nutrients, and radiological analytes in ground water from selected sites, Snake River Plain aquifer, south of the Idaho National Laboratory, Idaho: State of Idaho Department of Environmental Quality Division of INL Oversight and Radiation Control, OP-06-03, 28 p.
Helsel, D.R., Hirsch, R.M., Ryberg, K.R., Archfield, S.A., and Gilroy, E.J., 2020, Statistical methods in water resources: U.S. Geological Survey Techniques and Methods, book 4, chap. A3, 458 p. [Supersedes USGS Techniques of Water-Resources Investigations, book 4, chap. A3, version 1.1.] [Also available at https://doi.org/10.3133/tm4a3.]
Idaho Department of Environmental Quality, 2021, Idaho National Laboratory Oversight Program annual report: Department of Environmental Quality, 60 p. [Also available at https://www2.deq.idaho.gov/admin/LEIA/api/document/download/21821.]
Idaho Department of Environmental Quality, 2022a, Environmental Surveillance Program Quarterly Data Report: Idaho Oversight Program, July–September 2022. [Also available at https://www.deq.idaho.gov/idaho-national-laboratory-oversight/inl-oversight-program/monitoring-activities/.]
Idaho Department of Environmental Quality, 2022b, Environmental Surveillance Program Quarterly Data Report: Idaho Oversight Program, October–December 2022. [Also available at https://www.deq.idaho.gov/idaho-national-laboratory-oversight/inl-oversight-program/monitoring-activities/.]
Lewis, B.D., and Jensen, R.G., 1985, Hydrologic conditions at the Idaho National Engineering Laboratory, Idaho—1979–1981 update: U.S. Geological Survey Open-File Report 84–230 (IDO-22066), 65 p. [Also available at https://doi.org/10.3133/ofr85636.]
Litteer, D.L., 1988, Idaho National Engineering Laboratory radioactive waste management information for 1987: U.S. Department of Energy, Waste Management Programs Division, Idaho Operations Office Publication, DOE/ID-10055 (87), 530 p. [Also available at https://idahoenvironmental.com/ARIR/default.]
Litteer, D.L., and Reagan, B.D., 1989, Idaho National Engineering Laboratory radioactive waste management information for 1988: U.S. Department of Energy, Waste Management Programs Division, Idaho Operations Office Publication, DOE/ID-10055 (88), 510 p. [Also available at https://idahoenvironmental.com/ARIR/default.]
Maimer, N.V., and Bartholomay, R.C., 2019, Iodine-129 in the eastern Snake River Plain aquifer at and near the Idaho National Laboratory, Idaho, 2017–18: U.S. Geological Survey Scientific Investigations Report 2019–5133 (DOE/ID-22250), 20 p. [Also available at https://doi.org/10.3133/sir20195133.]
Mann, L.J., 1986, Hydraulic properties of rock units and chemical quality of water for INEL-1—A 10,365-foot deep test hole drilled at the Idaho National Engineering Laboratory, Idaho: U.S. Geological Survey Water-Resources Investigations Report 86–4020 (DOE/ID-22070), 23 p. [Also available at https://doi.org/10.3133/wri864020.]
Mann, L.J., and Beasley, T.M., 1994a, Background concentrations of (super 129) I in ground and surface water, eastern Snake River Plain, Idaho-1992: Journal of the Idaho Academy of Sciences, v. 30 no. 2, p. 75–87. [Also available at https://pubs.usgs.gov/publication/70180957.]
Mann, L.J., and Beasley, T.M., 1994b, Iodine-129 in the Snake River Plain aquifer at the Idaho National Engineering Laboratory, Idaho, 1990-1991: U.S. Geological Survey Water-Resources Investigations Report 94–4053 (DOE/ID-22115), 27 p. [Also available at https://doi.org/10.3133/wri944053.]
Mann, L.J., Chew, E.W., Morton, J.S., and Randolph, R.B., 1988, Iodine-129 in the Snake River Plain aquifer at the Idaho National Engineering Laboratory, Idaho: U.S. Geological Survey Water-Resources Investigations Report 88–4165 (DOE/ID-22076), 27 p. [Also available at https://doi.org/10.3133/wri884165.]
Maupin, M.A., and Barber, N.L., 2005, Estimated withdrawals from principal aquifers in the United States, 2000: U.S. Geological Survey Circular 1279, 46 p., accessed April 1, 2024, https://pubs.usgs.gov/circ/2005/1279. [Also available at https://doi.org/10.3133/cir1279.]
Mirus, B.B., Perkins, K.S., and Nimmo, J.R., 2011, Assessing controls on perched saturated zones beneath the Idaho Nuclear Technology and Engineering Center, Idaho: U.S. Geological Survey Scientific Investigations Report 2011–5222 (DOE/ID-22216), 20 p. [Also available at https://doi.org/10.3133/sir20115222.]
Neeway, J.J., Kaplan, D.I., Bagwell, C.E., Rockhold, M.L., Szecsody, J.E., Truex, M.J., Qafoku, N.P., 2019, A review of the behavior of radioiodine in the subsurface at two DOE sites: Science of the Total Environment, v. 691, p. 466–475. [Also available at https://doi.org/10.1016/j.scitotenv.2019.07.146.]
Nimmo, J.R., Perkins, K.S., Rose, P.A., Rousseau, J.P., Orr, B.R., Twining, B.V., and Anderson, S.R., 2002, Kilometer-scale rapid transport of naphthalene sulfonate tracer in the unsaturated zone at the Idaho National Engineering and Environmental Laboratory: Vadose Zone Journal, v. 1, p. 89–101. [Also available at https://doi.org/10.2113/1.1.89.]
Plummer, L.N., Rupert, M.G., Busenberg, E., and Schlosser, P., 2000, Age of irrigation water in ground water from the eastern Snake River Plain aquifer, south-central Idaho: Groundwater, v. 38, no. 2, p. 264–283. [Also available at https://doi.org/10.1111/j.1745-6584.2000.tb00338.x.]
Rattray, G.W., 2018, Geochemistry of groundwater in the eastern Snake River Plain aquifer, Idaho National Laboratory and vicinity, eastern Idaho: U.S. Geological Survey Professional Paper 1837-A (DOE/ID-22246), 198 p. [Also available at https://doi.org/10.3133/pp1837A.]
Rattray, G.W., 2023, Determining three-dimensional hydrologic processes in the eastern Snake River Plain aquifer using geochemical mass-balance modeling, Idaho National Laboratory, eastern Idaho, with contributions by Treinen, K.C.: U.S. Geological Survey Professional Paper 1837–C (DOE/ID-22258), 133 p. [Also available at https://doi.org/10.3133/pp1837C.]
R Core Team, 2024, R—A language and environment for statistical computing: Vienna, Austria, R Foundation for Statistical Computing, accessed on January 10, 2024, at https://www.r-project.org/.
Robertson, J.B., Schoen, R., and Barraclough, J.T., 1974, The influence of liquid waste disposal on the geochemistry of water at the National Reactor Testing Station, Idaho,1952–1970: U.S. Geological Survey Open-File Report 73–238 (IDO-22053), 231 p. [Also available at https://doi.org/10.3133/ofr73238.]
Rodgers, D.W., Ore, H.T., Bobo, R. T., McQuarrie, N, and Zentner, N., 2002, Extension and subsidence of the eastern Snake River Plain, Idaho, in Bill Bonnichsen, C.M. White, and Michael McCurry, eds., Tectonic and magmatic evolution of the Snake River Plain Volcanic Province: Idaho Geological Survey Bulletin 30, p. 121–155. [Also available at https://www.researchgate.net/publication/265996688.]
Sen, P.K., 1968, Estimates of the regression coefficient based on Kendall's tau: Journal of the American Statistical Association, v. 63, no. 324, p. 1379–1389. [Also available at https://doi.org/10.2307/2285891.]
Sharma, P., Bourgeois, M., Elmore, D., Granger, D., Lipschutz, M.E., Ma, X., Miller, T.E., Mueller, K.A., Rickey, F.A., Simms, P.C., and Vogt, S.K., 2000, PRIME lab AMS performance, upgrades, and research applications: Nuclear Instruments and Methods in Physics Research Section B—Beam Interactions with Materials and Atoms, v. 172, p. 112–123, accessed March 3, 2023, at https://doi.org/10.1016/S0168-583X(00)00132-4.
Schusler, K.L., Pearson, D.M., McCurry, M.J., Bartholomay, R.C., and Anders, M.H., 2020, Regionally continuous Miocene rhyolites beneath the eastern Snake River Plain reveal localized flexure at its western margin—Idaho National Laboratory and vicinity: The Mountain Geologist, v. 57, no. 3. [Also available at https://doi.org/10.31582/rmag.mg.57.3.241.].
Treinen, K.C., Trcka, A.R., and Fisher, J.C., 2024, An update of hydrologic conditions and distribution of selected constituents in water, eastern Snake River aquifer and perched groundwater zones, Idaho National Laboratory, Idaho, emphasis 2019–21: U.S. Geological Survey Scientific Investigations Report 2023–5128 (DOE/ID-22261), 96 p. [Also available at https://doi.org/10.3133/sir20235128.]
Twining, B.V., Bartholomay, R.C., Fisher, J.C., and Anderson, C., 2021, Multilevel groundwater monitoring of hydraulic head, water temperature, and chemical constituents in the eastern Snake River Plain aquifer, Idaho National Laboratory, Idaho, 2014–18: U.S. Geological Survey Scientific Investigations Report 2021–5002, 82 p. [Also available at https://doi.org/10.3133/sir20215002.]
Twining, B.V., and Maimer, N.V., 2019, Transmissivity and geophysical data for selected wells located at and near the Idaho National Laboratory, Idaho, 2017–18: U.S. Geological Survey Scientific Investigations Report 2019–5134 (DOE/ID–22249), 30 p. plus appendixes. [Also available at https://doi.org/10.3133/sir20195134.]
U.S. Department of Energy, 2004, Monitoring report decision summary for operable unit 3-13, group 5, Snake River Plain aquifer: U.S. Department of Energy Idaho Operations Office Publication, DOE/ID-11098, rev. 1, variously paginated. [Also available at https://idahoenvironmental.com/ARIR/default.]
U.S. Department of Energy, 2007, Waste area group 10, operable unit 10-08, remedial investigation/feasibility study annual status report for fiscal year 2006: U.S. Department of Energy Idaho Operations Office Publication, DOE/ID-11297, rev. 0, 245 p. [Also available at https://idahoenvironmental.com/ARIR/default.]
U.S. Department of Energy, 2008, Waste area group 10, operable unit 10-08, annual monitoring status report for fiscal year 2007: U.S. Department of Energy Idaho Operations Office Publication, DOE/ID-11355, rev. 0, 123 p. [Also available at https://idahoenvironmental.com/ARIR/default.]
U.S. Department of Energy, 2012, Fiscal year 2011 annual operations and maintenance report for operable unit 3-14, Tank Farm soil and INTEC groundwater: U.S. Department of Energy Idaho Operations Office Publication, DOE/ID-11462, rev. 0, 140 p. [Also available at https://idahoenvironmental.com/ARIR/default.]
U.S. Environmental Protection Agency, 2022, Part141—National Primary Drinking Water Regulations: Washington, D.C., Code of Federal Regulations 40, Office of the Federal Register, National Archives and Records Administration, 40 CFR Part 141, accessed July 1, 2022, at https://www.ecfr.gov/current/title-40/ chapter-I/subchapter-D/part-141.
U.S. Geological Survey, 2024, National Water Information System: U.S. Geological Survey web page, accessed February 1, 2023, at https://doi.org/10.5066/F7P55KJN.
U.S. Geological Survey, variously dated, National field manual for the collection of water-quality data: U.S. Geological Survey Techniques of Water-Resources Investigations, book 9, chap. A1–A9. [Also available at https://pubs.water.usgs.gov/twri9A.]
Williams, L.M., 1996, Evaluation of quality assurance/quality control data collected by the U.S. Geological Survey for water-quality activities at the Idaho National Engineering Laboratory, Idaho, 1989 through 1993: U.S. Geological Survey Water-Resources Investigations Report 96–4148 (DOE/ID-22129), 116 p. [Also available at https://doi.org/10.3133/wri964148.]
Wood, W.W., 1981, Guidelines for collection and field analysis of ground-water samples for selected unstable constituents: U.S. Geological Survey Techniques of Water-Resources Investigations, book 1, chapter D2, 24 p. [Also available at https://pubs.usgs.gov/publication/twri01D2.]
Conversion Factors
U.S. customary units to International System of Units
Multiply | By | To obtain |
---|---|---|
foot (ft) | 0.3048 | meter (m) |
mile (mi) | 1.609 | kilometer (km) |
square foot (ft2) | 0.09290 | square meter (m2) |
square mile (mi2) | 2.590 | square kilometer (km2) |
gallon (gal) | 3.785 | liter (L) |
picocurie per liter (pCi/L) | 0.037 | becquerel per liter (Bq/L) |
foot per day (ft/d) | 0.3048 | meter per day (m/d) |
foot per mile (ft/mi) | 0.1894 | meter per kilometer (m/km) |
foot squared per day (ft2/d) | 0.09290 | meter squared per day (m2/d) |
Temperature in degrees Celsius (°C) may be converted to degrees Fahrenheit (°F) as follows:
°F = (1.8 × °C) + 32.
Datums
Vertical coordinate information is referenced to the North American Vertical Datum of 1988 (NAVD 88).
Horizontal coordinate information is referenced to the North American Datum of 1983 (NAD 83).
Supplemental Information
Concentrations of chemical constituents in water are given in milligrams per liter (mg/L). Select data analysis units are provided as nanograms per liter (ng/L). Activities for radioactive constituents in water are given in picocuries per liter (pCi/L), which is equal to 0.037 becquerel per liter, or one radioactive decay per second.
Abbreviations
129I
Iodine-129
AMS
accelerator mass spectrometry
ATRC
Advanced Test Reactor Complex
CFA
Central Facilities Area
Ci
curie
DEQ
Department of Environmental Quality
DOE
U.S. Department of Energy
EPA
U.S. Environmental Protection Agency
ESRP
eastern Snake River Plain
INL
Idaho National Laboratory
INLOP
Idaho National Laboratory Oversight Program
INLPO
U.S. Geological Survey Idaho National Laboratory Project Office
INTEC
Idaho Nuclear Technology and Engineering Center
MCL
maximum contaminant level
MLMS
multilevel monitoring systems
PRIME
Purdue Rare Isotope Measurement Laboratory
QA
quality assurance
QC
quality control
R2
coefficient of determination
RWMC
Radioactive Waste Management Complex
TRA
Test Reactor Area
USGS
U.S. Geological Survey
For information about the research in this report, contact
Director, Idaho Water Science Center
U.S. Geological Survey
230 Collins Rd
Boise, Idaho 83702-4250
https://www.usgs.gov/centers/id-water
Manuscript approved on December 4, 2024
Publishing support provided by the U.S. Geological Survey
Science Publishing Network, Tacoma Publishing Service Center
Disclaimers
Any use of trade, firm, or product names is for descriptive purposes only and does not imply endorsement by the U.S. Government.
Although this information product, for the most part, is in the public domain, it also may contain copyrighted materials as noted in the text. Permission to reproduce copyrighted items must be secured from the copyright owner.
Suggested Citation
Treinen, K.C., Trcka, A.R., Krohe, N., and Lehotsky, G., 2024, Iodine-129 in the eastern Snake River Plain aquifer at and near the Idaho National Laboratory, Idaho, 2021–22: U.S. Geological Survey Scientific Investigations Report 2024–5124 (DOE/ID 22262), 27 p., https://doi.org/10.3133/sir20245124.
ISSN: 2328-0328 (online)
Study Area
Publication type | Report |
---|---|
Publication Subtype | USGS Numbered Series |
Title | Iodine-129 in the eastern Snake River Plain aquifer at and near the Idaho National Laboratory, Idaho, 2021–22 |
Series title | Scientific Investigations Report |
Series number | 2024-5124 |
DOI | 10.3133/sir20245124 |
Year Published | 2024 |
Language | English |
Publisher | U.S. Geological Survey |
Publisher location | Reston, VA |
Contributing office(s) | Idaho Water Science Center |
Description | Report: vii, 27 p.; Data Release |
Country | United States |
State | Idaho |
Other Geospatial | Eastern Snake River Plain aquifer |
Online Only (Y/N) | Y |